Why the Balance Principle Should Replace the Reynolds Transport Theorem
Total Page:16
File Type:pdf, Size:1020Kb
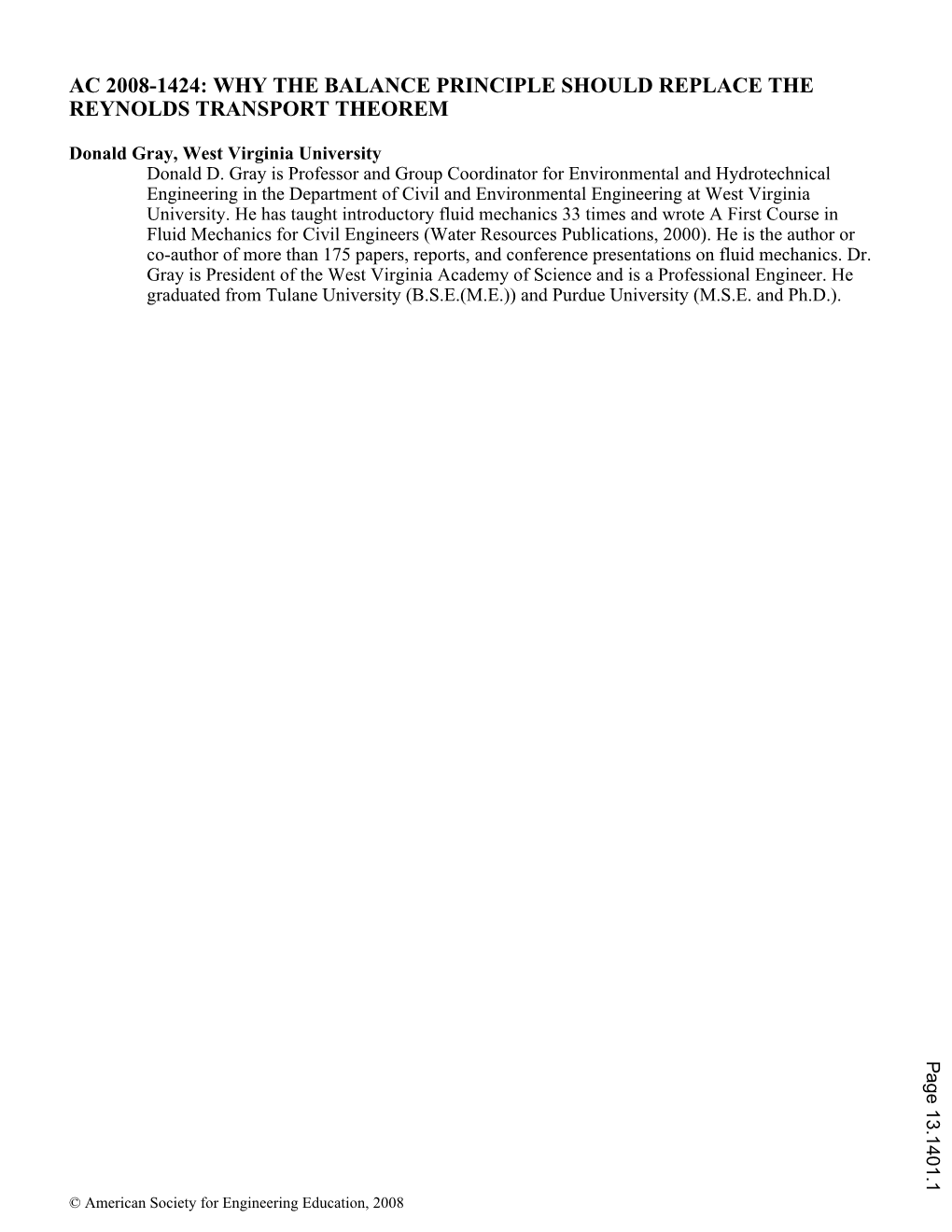
Load more
Recommended publications
-
Linear-Nonequilibrium Thermodynamics Theory for Coupled Heat and Mass Transport
University of Nebraska - Lincoln DigitalCommons@University of Nebraska - Lincoln Chemical and Biomolecular Research Papers -- Yasar Demirel Publications Faculty Authors Series 2001 Linear-nonequilibrium thermodynamics theory for coupled heat and mass transport Yasar Demirel University of Nebraska - Lincoln, [email protected] Stanley I. Sandler University of Delaware, [email protected] Follow this and additional works at: https://digitalcommons.unl.edu/cbmedemirel Part of the Chemical Engineering Commons Demirel, Yasar and Sandler, Stanley I., "Linear-nonequilibrium thermodynamics theory for coupled heat and mass transport" (2001). Yasar Demirel Publications. 8. https://digitalcommons.unl.edu/cbmedemirel/8 This Article is brought to you for free and open access by the Chemical and Biomolecular Research Papers -- Faculty Authors Series at DigitalCommons@University of Nebraska - Lincoln. It has been accepted for inclusion in Yasar Demirel Publications by an authorized administrator of DigitalCommons@University of Nebraska - Lincoln. Published in International Journal of Heat and Mass Transfer 44 (2001), pp. 2439–2451 Copyright © 2001 Elsevier Science Ltd. Used by permission. www.elsevier.comllocate/ijhmt Submitted November 5, 1999; revised September 6, 2000. Linear-nonequilibrium thermodynamics theory for coupled heat and mass transport Yasar Demirel and S. I. Sandler Center for Molecular and Engineering Thermodynamics, Department of Chemical Engineering, University of Delaware, Newark, DE 19716, USA Corresponding author — Y. Demirel Abstract Linear-nonequilibrium thermodynamics (LNET) has been used to express the entropy generation and dis- sipation functions representing the true forces and flows for heat and mass transport in a multicomponent fluid. These forces and flows are introduced into the phenomenological equations to formulate the cou- pling phenomenon between heat and mass flows. -
Control Volume Approach and Continuity Equation
Fluid Mechanics Chapter 5 Control Volume Approach and Continuity Equation Dr. Amer Khalil Ababneh Approach to Analyses of Fluid flows The engineer can find flow properties (pressure and velocity) in a flow field in one of two ways. One approach is to generate a series of pathlines or streamlines through the field and determine flow properties at any point along the lines by applying equations like those developed in Chapter 4. This is called the Lagrangian approach. The other way is to solve a set of equations for flow properties at any point in the flow field. This is called the Eulerian approach (also called control volume approach). The foundational concepts for the Eulerian approach, or control volume approach, are developed and applied to the conservation of mass. This leads to the continuity equation, a fundamental and widely used equation in fluid mechanics. Control volume is a region in space that allows mass to flow in and out of it. 5.1 Rate of Flow It is necessary to be able to calculate the flow rates through a control volume. Also, the capability to calculate flow rates is important in analyzing water supply systems, natural gas distribution networks, and river flows. Discharge The discharge, Q, often called the volume flow rate, is the volume of fluid that passes through an area per unit time. For example, when filling the gas tank of an automobile, the discharge or volume flow rate would be the gallons per minute flowing through the nozzle. Typical units for discharge are ft3/s (cfs), ft3/min (cfm), gpm, m3/s, and L/s. -
1 IV. First Law of Thermodynamics IV. First Law of Thermodynamics
IV. First Law of Thermodynamics A. Introduction to Open Systems 1. Analysis of flow processes begins with the selection of an open system. 2. An open system is a region of space called a control volume (CV). Mass Mass Control entering leaving volume (inlet) (exit) CV Q W lesson 11 IV. First Law of Thermodynamics 3. Example We can write a balance equation for the conservation of any extensive property of the open system. As an example, consider the mass of water in the Great Salt Lake. We might be interested in (1) the instantaneous rate of change of mass or (2) the change that occurs over a period of time. ⎛rate of change ⎞ ⎛rate at which⎞ ⎛rate at which⎞ ⎜ ⎟ ⎜ ⎟ ⎜ ⎟ ⎜of mass of water ⎟ = ⎜ water enters ⎟ − ⎜ water leaves⎟ (1) ⎜ ⎟ ⎜ ⎟ ⎜ ⎟ ⎝in lake ⎠ ⎝lake ⎠ ⎝lake ⎠ ⎛change in mass ⎞ ⎛amount of water ⎞ ⎛amount of water ⎞ ⎜ ⎟ ⎜ ⎟ ⎜ ⎟ ⎜of water in lake ⎟ = ⎜ which enters lake⎟ − ⎜which leaves lake⎟ (2) ⎜ ⎟ ⎜ ⎟ ⎜ ⎟ ⎝during February⎠ ⎝during February ⎠ ⎝during February ⎠ We obtain Form (2) by integrating Form (1). We use both forms for mass, energy, and entropy. lesson 11 1 IV. First Law of Thermodynamics B. Conservation of Mass for a Control Volume 1. For a closed system, msys = constant and dmsys/dt = 0 2. For an open system ⎛Time rate of change of ⎞ ⎛total rate of mass⎞ ⎛total rate of mass⎞ ⎜ ⎟ ⎜ ⎟ ⎜ ⎟ ⎜mass within a control ⎟ = ⎜entering a control ⎟ − ⎜leaving a control ⎟ ⎜ ⎟ ⎜ ⎟ ⎜ ⎟ ⎝volume at time t ⎠ ⎝volume at time t ⎠ ⎝volume at time t ⎠ dmCV =−∑mm∑ (kg/s) (5-9) dt in out (5-9) can be integrated with respect to time to give mmm ∆ CV =−∑ ∑ (kg) (5-8) in out lesson 11 IV. -
Nonequilibrium Thermodynamics of Porous Electrodes
Nonequilibrium Thermodynamics of Porous Electrodes The MIT Faculty has made this article openly available. Please share how this access benefits you. Your story matters. Citation Ferguson, T. R., and M. Z. Bazant. “Nonequilibrium Thermodynamics of Porous Electrodes.” Journal of the Electrochemical Society 159.12 (2012): A1967–A1985. © 2012 The Electrochemical Society As Published http://dx.doi.org/10.1149/2.048212jes Publisher The Electrochemical Society Version Final published version Citable link http://hdl.handle.net/1721.1/77923 Terms of Use Article is made available in accordance with the publisher's policy and may be subject to US copyright law. Please refer to the publisher's site for terms of use. Nonequilibrium Thermodynamics of Porous Electrodes Todd R. Ferguson and Martin Z. Bazant J. Electrochem. Soc. 2012, Volume 159, Issue 12, Pages A1967-A1985. doi: 10.1149/2.048212jes Email alerting Receive free email alerts when new articles cite this article - sign up service in the box at the top right corner of the article or click here To subscribe to Journal of The Electrochemical Society go to: http://jes.ecsdl.org/subscriptions © 2012 The Electrochemical Society Journal of The Electrochemical Society, 159 (12) A1967-A1985 (2012) A1967 0013-4651/2012/159(12)/A1967/19/$28.00 © The Electrochemical Society Nonequilibrium Thermodynamics of Porous Electrodes Todd R. Fergusona and Martin Z. Bazanta,b,∗,z aDepartment of Chemical Engineering, Massachusetts Institute of Technology, Cambridge, Massachusetts 02139, USA bDepartment of Mathematics, Massachusetts Institute of Technology, Cambridge, Massachusetts 02139, USA We reformulate and extend porous electrode theory for non-ideal active materials, including those capable of phase transformations. -
Fundamental Laws of Motion for Particles, Material Volumes, and Control Volumes
1 Fundamental Laws of Motion for Particles, Material Volumes, and Control Volumes Ain A. Sonin Department of Mechanical Engineering Massachusetts Institute of Technology Cambridge, MA 02139, USA August 2001 © Ain A. Sonin Contents 1 Basic laws for material volumes 2 Material volumes and material particles 2 Laws for material particles 3 Mass conservation 3 Newton’s law of (non-relativistic) linear motion 3 Newton’s law applied to angular momentum 4 First law of thermodynamics 4 Second law of thermodynamics 5 Laws for finite material volumes 5 Mass conservation 5 Motion (linear momentum) 6 Motion (angular momentum) 6 First law of thermodynamics 7 Second law of thermodynamics 8 2 The transformation to control volumes 8 The control volume 8 Rate of change over a volume integral over a control volume 9 Rate of change of a volume integral over a material volume 10 Reynolds’ material-volume to control-volume transformation 11 3 Basic laws for control volumes 13 Mass conservation 13 Linear momentum theorem 14 Angular momentum theorem 15 First law of thermodynamics 16 Second law of thermodynamics 16 4 Procedure for control volume analysis 17 2 1 Basic Laws for Material Volumes Material volumes and material particles The behavior of material systems is controlled by universal physical laws. Perhaps the most ubiquitous of these are the law of mass conservation, the laws of motion published by Isaac Newton's in 1687, and the first and second laws of thermodynamics, which were understood before the nineteenth century ended. In this chapter we will review these four laws, starting with their most basic forms, and show how they can be expressed in forms that apply to control volumes. -
The First Law of Thermodynamics for Control Volumes Part I
The First Law of Thermodynamics for Control Volumes Part I The Energy Equation for Control Volumes In this course we consider three types of Control Volume Systems - Steam Power Plants, Refrigeration Systems, and Aircraft Jet Engines. Fortunately we will be able to separately analyse each component of the system independent of the entire system, which is typically represented as follows: In addition to the energy flow across the control volume boundary in the form of heat and work, we will also have mass flowing into and out of the control volume. We will only consider Steady Flow conditions throughout, in which there is no energy or mass accumulation in the control volume, thus we will find it convenient to derive the energy equation in terms of power [kW] rather than energy [kJ]. Furthermore the term Control Volume indicates that there is no boundary work done by the system, and typically we have shaft work, such as with a turbine, compressor or pump. Mass Flow Consider an elemental mass dm flowing through an inlet or outlet port of a control volume, having an area A, volume dV, length dx, and an average steady velocity , as follows. Thus finally the mass flow rate can be determined as follows: Flow Energy The fluid mass flows through the inlet and exit ports of the control volume accompanied by its energy. These include four types of energy - internal energy (u), kinetic enegy (ke), potential energy (pe), and flow work (wflow). In order to evaluate the flow work consider the following exit port schematic showing the fluid doing work against the surroundings through an imaginary piston: It is of interest that the specific flow work is simply defined by the pressure P multiplied by the specific volume v. -
Chapter 5 Finite Control Volume Analysis
57:020 Mechanics of Fluids and Transport Processes Chapter 5 Professor Fred Stern Fall 2009 1 Chapter 5 Finite Control Volume Analysis 5.1 Continuity Equation RTT can be used to obtain an integral relationship expressing conservation of mass by defining the extensive property B = M such that β = 1. B = M = mass β = dB/dM = 1 General Form of Continuity Equation dM d = 0 = ∫∫ρdV + ρV ⋅dA dt dt CV CS or d ∫ρV ⋅dA = − ∫ρdV CS dt CV net rate of outflow rate of decrease of of mass across CS mass within CV Simplifications: d 1. Steady flow: − ∫ρdV = 0 dt CV 2. V = constant over discrete dA (flow sections): ∫ ρV ⋅dA = ∑ρV ⋅A CS CS 57:020 Mechanics of Fluids and Transport Processes Chapter 5 Professor Fred Stern Fall 2009 2 3. Incompressible fluid (ρ = constant) d ∫∫VdA⋅=− dV conservation of volume CSdt CV 4. Steady One-Dimensional Flow in a Conduit: ∑ρV ⋅A = 0 CS −ρ1V1A1 + ρ2V2A2 = 0 for ρ = constant Q1 = Q2 Some useful definitions: Mass flux m& = ∫ρV ⋅dA A Volume flux Q = ∫ V ⋅dA A Average Velocity V = Q / A 1 Average Density ρ = ∫ρdA A Note: mQ& ≠ ρ unless ρ = constant 57:020 Mechanics of Fluids and Transport Processes Chapter 5 Professor Fred Stern Fall 2009 3 Example *Steady flow *V1,2,3 = 50 fps *@ © V varies linearly from zero at wall to Vmax at pipe center *find m& 4, Q4, Vmax 3 0 *water, ρw = 1.94 slug/ft d ∫ρV ⋅dA = 0 = − ∫ρdV dt CS CV m& 4 ρ ρ ρ ρ i.e., - 1V1A1 - 2V2A2 + 3V3A3 + ∫ V4dA4 = 0 A4 ρ = const. -
Thermodynamics
Thermodynamics What is it? Why do we study it? Thermodynamics Science dealing with how Matter behaves in relation to heat and work exchanged with the surroundings. Thermodynamics Science dealing with how Matter behaves in relation to heat and work exchanged with the surroundings. Phases of Matter - Solid (ES2001 Materials), Liquids (ES3001), Gases (ES3001), Plasma (Graduate School) Applications of Thermodynamics: (See Table 1.1 of text - huge!) Thermodynamics The Design and Analysis of many engineering systems requires thermodynamics (in addition to fluid mechanics, heat transfer, structural analysis, etc.). Another short description of Thermodynamics is: Link Location: http://www.sciencelearn.org.nz/Science-Stories/Science-Made-Simple/Sci-Media/Video/Thermodynamics Course Objectives: Thermodynamics is both a branch of science and a specialty within engineering. In this course you will be introduced to properties of matter (Temperature, Pressure, Enthalpy, Specific Volume, Entropy, etc.) and governing laws which can be used to describe the behavior of matter and its interaction with the surrounding environment. Course Objectives: Thermodynamics is both a branch of science and a specialty within engineering. In this course you will be introduced to properties of matter (Temperature, Pressure, Enthalpy, Specific Volume, Entropy, etc.) and governing laws which can be used to describe the behavior of matter and its interaction with the surrounding environment. You will learn how to identify systems and to use thermodynamic analysis to describe the behavior of the system in terms of properties and processes. Understanding how to apply these concepts is a powerful tool for the engineer, enabling the evaluation of material states (phases) under different conditions and the maximum efficiency achievable with various power cycles. -
The Rate-Controlled Constrained-Equilibrium Approach to Far-From-Local-Equilibrium Thermodynamics
Entropy 2012, 14, 92-130; doi:10.3390/e14020092 OPEN ACCESS entropy ISSN 1099-4300 www.mdpi.com/journal/entropy Article The Rate-Controlled Constrained-Equilibrium Approach to Far-From-Local-Equilibrium Thermodynamics Gian Paolo Beretta 1;?, James C. Keck 2;y, Mohammad Janbozorgi 3 and Hameed Metghalchi 3 1 Department of Mechanical and Industrial Engineering, Universita` di Brescia, Brescia, 25123, Italy 2 Department of Mechanical Engineering, Massachusetts Institute of Technology, Cambridge, MA 02139, USA 3 Department of Mechanical Engineering, Northeastern University Boston, MA 02115, USA; E-Mails: [email protected] (M.J.); [email protected] (H.M.) y Deceased. ? Author to whom correspondence should be addressed; E-Mail: [email protected]. Received: 12 October 2011; in revised form: 31 December 2011 / Accepted: 18 January 2012 / Published: 30 January 2012 Abstract: The Rate-Controlled Constrained-Equilibrium (RCCE) method for the description of the time-dependent behavior of dynamical systems in non-equilibrium states is a general, effective, physically based method for model order reduction that was originally developed in the framework of thermodynamics and chemical kinetics. A generalized mathematical formulation is presented here that allows including nonlinear constraints in non-local equilibrium systems characterized by the existence of a non-increasing Lyapunov functional under the system’s internal dynamics. The generalized formulation of RCCE enables to clarify the essentials of the method and the built-in general feature of thermodynamic consistency in the chemical kinetics context. In this paper, we work out the details of the method in a generalized mathematical-physics framework, but for definiteness we detail its well-known implementation in the traditional chemical kinetics framework. -
The Energy Equation for Control Volumes Recall, the First Law Of
The Energy Equation for Control Volumes Recall, the First Law of Thermodynamics: where = rate of change of total energy of the system, = rate of heat added to the system, = rate of work done by the system In the Reynolds Transport Theorem (R.T.T.), let . So, The left side of the above equation applies to the system, and the right side corresponds to the control volume. Thus, the right side of the above equation can be called the General Integral Equation for Conservation of Energy in a Control Volume, where e = total energy of the fluid per unit mass, , = internal energy per unit mass, = kinetic energy per unit mass, gz = potential energy per unit mass. Generally, what is done is to split the work term up into 3 parts: , where: = rate of shaft work, = rate of pressure work, = rate of viscous work. Note: has dimensions of {work/time} = Power (so these are actually power terms). Let's look at each of these terms individually: Rate of Shaft Work, = rate of work done by the fluid on a shaft protruding outside the C.V. E.g. a turbine (extracts energy from a flow) : The turbine takes energy from the fluid and converts it into rotation of the shaft. Note: is positive for a turbine (work done by the fluid), and is negative for a fan (work done on the fluid). Rate of Pressure Work, = rate of work done by pressure forces at the control surface It turns out that . Rate of viscous work, = rate of work done by viscous stresses at the control surface. -
Fluids – Lecture 9 Notes 1
Fluids – Lecture 9 Notes 1. Momentum-Integral Simplifications 2. Applications Reading: Anderson 2.6 Simplifications For steady flow, the momentum integral equation reduces to the following. ρ V~ ·nˆ V~ dA = −p nˆ dA + ρ~gdV + F~viscous (1) ZZ ZZ ZZZ Defining h as the height above ground, we note that ∇h is a unit vector which points up, so that the gravity acceleration vector can be written as a gradient. ~g = −g ∇h Using the Gradient Theorem, this then allows the gravity-force volume integral to be con- verted to a surface integral, provided we make the additional assumption that ρ is nearly constant throughout the flow. ρ~gdV = −ρg ∇hdV = −ρgh nˆ dA ZZZ ZZZ ZZ We can now combine the pressure and gravity contributions into one surface integral. −p nˆ dA + ρ~gdV → −(p + ρgh)ˆn dA ZZ ZZZ ZZ Defining a corrected pressure pc = p+ρgh, the Integral Momentum Equation finally becomes ρ V~ ·nˆ V~ dA = −pc nˆ dA + F~viscous (2) ZZ ZZ Aerodynamic analyses using (2) do not have to concern themselves with the effects of gravity, since it does not appear explicitly in this equation. In particular, the velocity field V~ will not be affected by gravity. Gravity enters the problem only in a secondary step, when the true pressure field p is constructed from pc by adding the “tilting” bias −ρgh. h h h h = + g ρ p pc g h net aerodynamic aerostatic (gravity neglected) (gravity alone) 1 For clarity, we will from now on refer to pc simply as p. -
Integral Relations for a Control Volume T Arayyes Introduction • in Analysing Fluid Motion, We Might Take One of Two Paths: 1
Integral Relations for a Control Volume T Arayyes Introduction • In analysing fluid motion, we might take one of two paths: 1. Seeking to describe the detailed flow pattern at every point (x, y, z) in the field OR 2. working with a finite region, making a balance of flow in versus flow out, and determining gross flow effects such as the force or torque on a body or the total energy exchange • The second is the “control-volume” method and is the subject of this chapter. • The first is the “differential” approach and is developed in Chap. 4.is • Control volume is a very quick analysis that give a quantitative results. • In Fluids: control volume used for conservation of mass, linear momentum, angular momentum, and energy • In thermodynamics it is mainly conservation of mass and energy. What is a control volume? • control volume approach: is to consider a fixed interior volume of a system • System approach: We follow the fluid as it moves and deforms.—no mass crosses the boundary, and the total mass of the system remains fixed. • an arbitrary quantity of mass of fixed identity. Everything external to this system is denoted by the term surroundings, • • Control volumes can be Fixed, moving, and deformable a) Fixed control volume for nozzle-stress analysis; b) Control volume moving at ship speed for drag-force analysis; c) Control volume deforming within cylinder for transient pressure- variation analysis. Review, Basic laws for a fixed mass or system approach • Conservation of mass: 푑푚 = 0 푑푡 Mass of the system can be defined as: 푀 = 푑푚 = 휌푑푉 푉(푠푦푠푡푒푚) • Conservation of Linear momentum, p: 푝 = 푚푣 푑푝 F = = 푚 푣 = 푚푎 (time rate of change of momentum) 푑푡 Linear momentum: 푝 = (푠푦푠) 푣 푑푚= 푉(푠푦푠) 푣 휌푑푉 • Conservation of Energy 훿푄 + 훿푊 = 푑퐸 Where Q is hear and W is work 푑퐸 푄 + 푊 = 푑푡 푠푦푠푡푒푚 퐸푠푦푠푡푒푚 = 푒.