Enhanced Group II Intron Retrohoming in Magnesium-Deficient Escherichia Coli Via Selection of Mutations in the Ribozyme Core
Total Page:16
File Type:pdf, Size:1020Kb
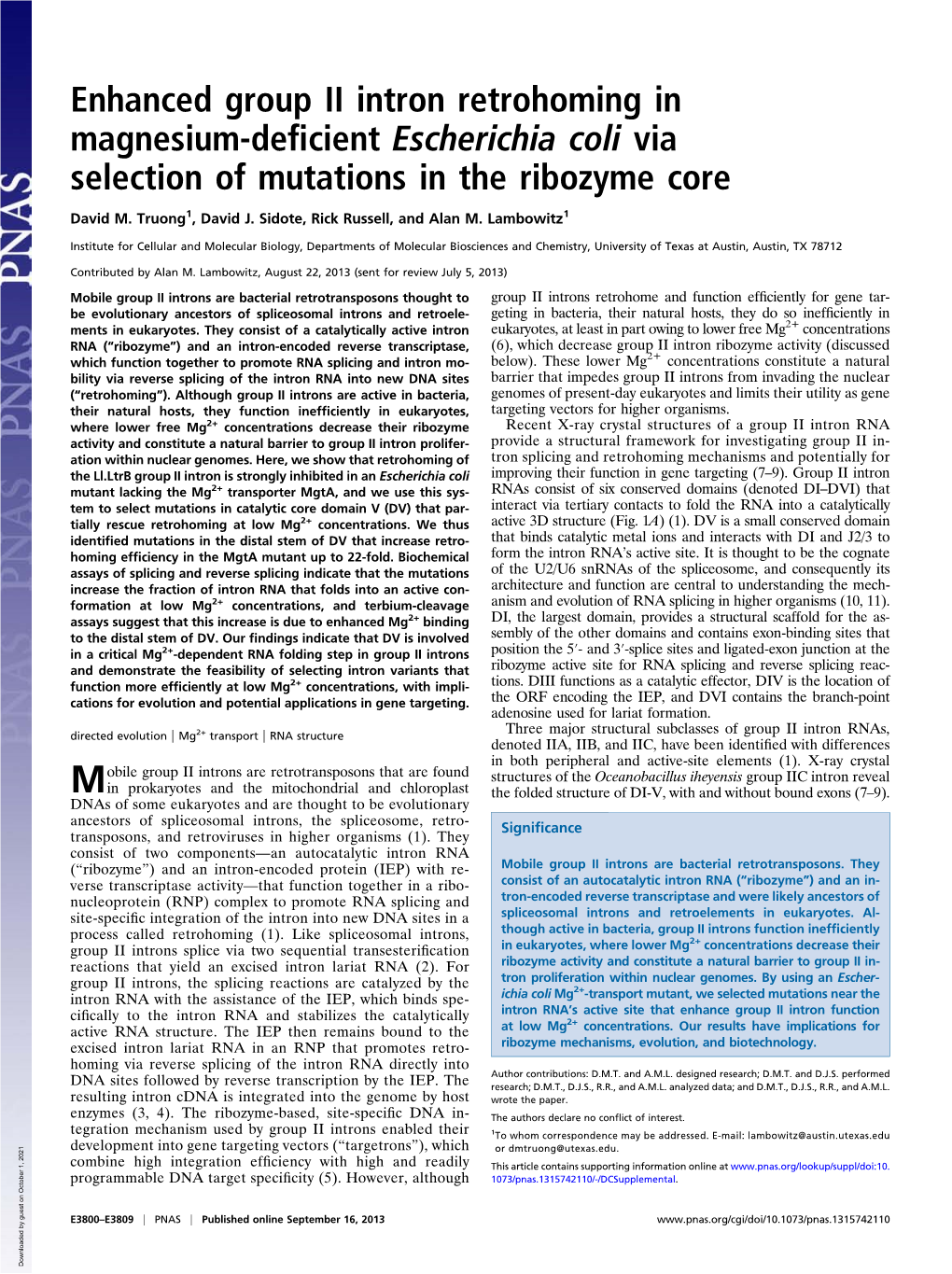
Load more
Recommended publications
-
Exploring the Structure of Long Non-Coding Rnas, J
IMF YJMBI-63988; No. of pages: 15; 4C: 3, 4, 7, 8, 10 1 2 Rise of the RNA Machines: Exploring the Structure of 3 Long Non-Coding RNAs 4 Irina V. Novikova, Scott P. Hennelly, Chang-Shung Tung and Karissa Y. Sanbonmatsu Q15 6 Los Alamos National Laboratory, Los Alamos, NM 87545, USA 7 Correspondence to Karissa Y. Sanbonmatsu: [email protected] 8 http://dx.doi.org/10.1016/j.jmb.2013.02.030 9 Edited by A. Pyle 1011 12 Abstract 13 Novel, profound and unexpected roles of long non-coding RNAs (lncRNAs) are emerging in critical aspects of 14 gene regulation. Thousands of lncRNAs have been recently discovered in a wide range of mammalian 15 systems, related to development, epigenetics, cancer, brain function and hereditary disease. The structural 16 biology of these lncRNAs presents a brave new RNA world, which may contain a diverse zoo of new 17 architectures and mechanisms. While structural studies of lncRNAs are in their infancy, we describe existing 18 structural data for lncRNAs, as well as crystallographic studies of other RNA machines and their implications 19 for lncRNAs. We also discuss the importance of dynamics in RNA machine mechanism. Determining 20 commonalities between lncRNA systems will help elucidate the evolution and mechanistic role of lncRNAs in 21 disease, creating a structural framework necessary to pursue lncRNA-based therapeutics. 22 © 2013 Published by Elsevier Ltd. 24 23 25 Introduction rather than the exception in the case of eukaryotic 50 organisms. 51 26 RNA is primarily known as an intermediary in gene LncRNAs are defined by the following: (i) lack of 52 11 27 expression between DNA and proteins. -
A Group Ill Twintron Encoding a Maturase-Like Gene Excises Through Lariat Intermediates
I.=) 1994 Oxford University Press Nucleic Acids Research, 1994, Vol. 22, No. 6 1029-1036 A group Ill twintron encoding a maturase-like gene excises through lariat intermediates Donald W.Copertino1 2+, Edina T.Hall2,§, Fredrick W.Van Hook2, Kristin P.Jenkins2 and Richard B.Hallick1,2* 1Departments of Biochemistry and 2Molecular and Cellular Biology, University of Arizona, Tucson, AZ 85721, USA Received December 9, 1993; Revised and Accepted February 10, 1994 ABSTRACT The 1605 bp intron 4 of the Euglena gracilis chloroplast the closely related nonphotosynthetic euglenoid Astasia longa psbC gene was characterized as a group Ill twintron (8, 9). composed of an internal 1503 nt group Ill intron with Euglena chloroplast genes also contain 15 twintrons, introns- an open reading frame of 1374 nt (ycfl3, 458 amino within-introns, that are sequentially spliced (3). There are acids), and an external group Ill intron of 102 nt. twintrons with one intron internal to another intron (2, 6, 10), Twintron excision proceeds by a sequential splicing and complex twintrons with multiple internal introns (11; for pathway. The splicing of the internal and external group review, see 3). Group II and group III introns can occur as Ill introns occurs via lariat intermediates. Branch sites internal and/or external introns of twintrons. Excision of internal were mapped by primer extension RNA sequencing. introns occurs prior to excision of external introns. The excision The unpaired adenosines in domains VI of the internal of internal group IH introns of twintrons can proceed from and external introns are covalently linked to the 5' multiple 5' and 3' splice sites (6, 1 1). -
The Association of Group IIB Intron with Integrons in Hypersaline Environments Sarah Sonbol1 and Rania Siam1,2*
Sonbol and Siam Mobile DNA (2021) 12:8 https://doi.org/10.1186/s13100-021-00234-2 RESEARCH Open Access The association of group IIB intron with integrons in hypersaline environments Sarah Sonbol1 and Rania Siam1,2* Abstract Background: Group II introns are mobile genetic elements used as efficient gene targeting tools. They function as both ribozymes and retroelements. Group IIC introns are the only class reported so far to be associated with integrons. In order to identify group II introns linked with integrons and CALINS (cluster of attC sites lacking a neighboring integron integrase) within halophiles, we mined for integrons in 28 assembled metagenomes from hypersaline environments and publically available 104 halophilic genomes using Integron Finder followed by blast search for group II intron reverse transcriptases (RT)s. Results: We report the presence of different group II introns associated with integrons and integron-related sequences denoted by UHB.F1, UHB.I2, H.ha.F1 and H.ha.F2. The first two were identified within putative integrons in the metagenome of Tanatar-5 hypersaline soda lake, belonging to IIC and IIB intron classes, respectively at which the first was a truncated intron. Other truncated introns H.ha.F1 and H.ha.F2 were also detected in a CALIN within the extreme halophile Halorhodospira halochloris, both belonging to group IIB introns. The intron-encoded proteins (IEP) s identified within group IIB introns belonged to different classes: CL1 class in UHB.I2 and bacterial class E in H.ha.Fa1 and H.ha.F2. A newly identified insertion sequence (ISHahl1)ofIS200/605 superfamily was also identified adjacent to H. -
Mutualism Between Self‑Splicing Introns and Their Hosts David R Edgell1*, Venkata R Chalamcharla2,3 and Marlene Belfort2*
Edgell DR et al. BMC Biology 2011, 9:22 http://www.biomedcentral.com/1741-7007/9/22 review Open Access Learning to live together: mutualism between self‑splicing introns and their hosts David R Edgell1*, Venkata R Chalamcharla2,3 and Marlene Belfort2* Abstract data argue that the relationship is more elaborate than previously appreciated. Group I and II introns can be considered as molecular parasites that interrupt protein-coding and structural Mobile introns: ribozymes with baggage RNA genes in all domains of life. They function as self- One group of mobile genetic elements comprises the group I splicing ribozymes and thereby limit the phenotypic and II introns. These sequences interrupt protein-coding and costs associated with disruption of a host gene while structural RNA genes in all domains of life and can be con they act as mobile DNA elements to promote their sidered as molecular parasites. When the gene is transcribed spread within and between genomes. Once considered into RNA, the intron sequence acts as a ribozyme (an RNA purely selfish DNA elements, they now seem, in the with enzymatic activity), which removes the intron sequence light of recent work on the molecular mechanisms from the primary RNA transcript, thus limiting the regulating bacterial and phage group I and II intron phenotypic cost associated with insertion of the element into dynamics, to show evidence of co-evolution with their a host gene and promoting their maintenance in the genome. hosts. These previously underappreciated relationships In the case of group I and II introns, the host-parasite serve the co-evolving entities particularly well in times relationship is enriched by the fact that the introns of environmental stress. -
Group II Intron Rnps and Reverse Transcriptases: from Retroelements to Research Tools
Downloaded from http://cshperspectives.cshlp.org/ on September 29, 2021 - Published by Cold Spring Harbor Laboratory Press Group II Intron RNPs and Reverse Transcriptases: From Retroelements to Research Tools Marlene Belfort1 and Alan M. Lambowitz2 1Department of Biological Sciences and RNA Institute, University at Albany, State University of New York, Albany, New York 12222 2Institute for Cellular and Molecular Biology and Department of Molecular Biosciences, University of Texas at Austin, Austin, Texas 78712 Correspondence: [email protected]; [email protected] SUMMARY Group II introns, self-splicing retrotransposons, serve as both targets of investigation into their structure, splicing, and retromobility and a source of tools for genome editing and RNA anal- ysis. Here, we describe the first cryo-electron microscopy (cryo-EM) structure determination, at 3.8–4.5 Å, of a group II intron ribozyme complexed with its encoded protein, containing a reverse transcriptase (RT), required for RNA splicing and retromobility. We also describe a method called RIG-seq using a retrotransposon indicator gene for high-throughput integration profiling of group II introns and other retrotransposons. Targetrons, RNA-guided gene targeting agents widely used for bacterial genome engineering, are described next. Finally, we detail thermostable group II intron RTs, which synthesize cDNAs with high accuracy and processiv- ity, for use in various RNA-seq applications and relate their properties to a 3.0-Å crystal structure of the protein poised for reverse transcription. Biological insights from these group II intron revelations are discussed. Outline 1 Introduction 5 Technique 4. RTs with novel enzymatic properties as potent research tools 2 Technique 1. -
The Chloroplast Trans-Splicing RNA–Protein Supercomplex from the Green Alga Chlamydomonas Reinhardtii
cells Review The Chloroplast Trans-Splicing RNA–Protein Supercomplex from the Green Alga Chlamydomonas reinhardtii Ulrich Kück * and Olga Schmitt Allgemeine und Molekulare Botanik, Faculty for Biology and Biotechnology, Ruhr-Universität Bochum, Universitätsstraße 150, 44780 Bochum, Germany; [email protected] * Correspondence: [email protected]; Tel.: +49-234-32-28951 Abstract: In eukaryotes, RNA trans-splicing is a significant RNA modification process for the end-to- end ligation of exons from separately transcribed primary transcripts to generate mature mRNA. So far, three different categories of RNA trans-splicing have been found in organisms within a diverse range. Here, we review trans-splicing of discontinuous group II introns, which occurs in chloroplasts and mitochondria of lower eukaryotes and plants. We discuss the origin of intronic sequences and the evolutionary relationship between chloroplast ribonucleoprotein complexes and the nuclear spliceosome. Finally, we focus on the ribonucleoprotein supercomplex involved in trans-splicing of chloroplast group II introns from the green alga Chlamydomonas reinhardtii. This complex has been well characterized genetically and biochemically, resulting in a detailed picture of the chloroplast ribonucleoprotein supercomplex. This information contributes substantially to our understanding of the function of RNA-processing machineries and might provide a blueprint for other splicing complexes involved in trans- as well as cis-splicing of organellar intron RNAs. Keywords: group II intron; trans-splicing; ribonucleoprotein complex; chloroplast; Chlamydomonas reinhardtii Citation: Kück, U.; Schmitt, O. The Chloroplast Trans-Splicing RNA–Protein Supercomplex from the Green Alga Chlamydomonas reinhardtii. 1. Introduction Cells 2021, 10, 290. https://doi.org/ One of the unexpected and outstanding discoveries in 20th century biology was the 10.3390/cells10020290 identification of discontinuous eukaryotic genes [1,2]. -
Group II Intron
Downloaded from rnajournal.cshlp.org on September 26, 2021 - Published by Cold Spring Harbor Laboratory Press Tertiary architecture of the Oceanobacillus iheyensis group II intron NAVTEJ TOOR,1,5 KEVIN S. KEATING,2 OLGA FEDOROVA,1 KANAGALAGHATTA RAJASHANKAR,3 JIMIN WANG,1 and ANNA MARIE PYLE1,4 1Department of Molecular Biophysics and Biochemistry, Yale University, New Haven, Connecticut 06511, USA 2Interdepartmental Program in Computational Biology and Bioinformatics, Yale University, New Haven, Connecticut 06511, USA 3The Northeastern Collaborative Access Team (NE-CAT) at the Advanced Photon Source at Argonne National Laboratory, Argonne, Illinois 60439, USA 4Howard Hughes Medical Institute, Chevy Chase, Maryland 20815, USA ABSTRACT Group II introns are large ribozymes that act as self-splicing and retrotransposable RNA molecules. They are of great interest because of their potential evolutionary relationship to the eukaryotic spliceosome, their continued influence on the organization of many genomes in bacteria and eukaryotes, and their potential utility as tools for gene therapy and biotechnology. One of the most interesting features of group II introns is their relative lack of nucleobase conservation and covariation, which has long suggested that group II intron structures are stabilized by numerous unusual tertiary interactions and backbone-mediated contacts. Here, we provide a detailed description of the tertiary interaction networks within the Oceanobacillus iheyensis group IIC intron, for which a crystal structure was recently solved to 3.1 A˚ resolution. The structure can be described as a set of several intricately constructed tertiary interaction nodes, each of which contains a core of extended stacking networks and elaborate motifs. Many of these nodes are surrounded by a web of ribose zippers, which appear to further stabilize local structure. -
Group II Intron-Mediated Trans-Splicing in the Gene-Rich Mitochondrial Genome of an Enigmatic Eukaryote, Diphylleia Rotans
View metadata, citation and similar papers at core.ac.uk brought to you by CORE provided by Tsukuba Repository Group II Intron-Mediated Trans-Splicing in the Gene-Rich Mitochondrial Genome of an Enigmatic Eukaryote, Diphylleia rotans 著者 Kamikawa Ryoma, Shiratori Takashi, Ishida Ken-Ichiro, Miyashita Hideaki, Roger Andrew J. journal or Genome biology and evolution publication title volume 8 number 2 page range 458-466 year 2016-02 権利 (C) The Author 2016. Published by Oxford University Press on behalf of the Society for Molecular Biology and Evolution. This is an Open Access article distributed under the terms of the Creative Commons Attribution Non-Commercial License (http://creativecommons.org/licenses/by-nc/4.0 /), which permits non-commercial re-use, distribution, and reproduction in any medium, provided the original work is properly cited. For commercial re-use, please contact [email protected] URL http://hdl.handle.net/2241/00138505 doi: 10.1093/gbe/evw011 Creative Commons : 表示 - 非営利 http://creativecommons.org/licenses/by-nc/3.0/deed.ja GBE Group II Intron-Mediated Trans-Splicing in the Gene-Rich Mitochondrial Genome of an Enigmatic Eukaryote, Diphylleia rotans Ryoma Kamikawa1,2,*, Takashi Shiratori3,Ken-IchiroIshida4, Hideaki Miyashita1,2, and Andrew J. Roger5,6 1Graduate School of Human and Environmental Studies, Kyoto University, Japan 2Graduate School of Global Environmental Studies, Kyoto University, Japan 3Graduate School of Life and Environmental Sciences, University of Tsukuba, Ibaraki, Japan 4Faculty of Life and Environmental Sciences, University of Tsukuba, Ibaraki, Japan 5 Centre for Comparative Genomics and Evolutionary Bioinformatics, Department of Biochemistry and Molecular Biology, Dalhousie University, Downloaded from Halifax, Nova Scotia, Canada 6Program in Integrated Microbial Biodiversity, Canadian Institute for Advanced Research, Halifax, Nova Scotia, Canada *Corresponding author: E-mail: [email protected]. -
Group II Intron Retroelements: Function and Diversity
Diversity of Retrotransposable Elements Cytogenet Genome Res 110:589–597 (2005) DOI: 10.1159/000084992 Group II intron retroelements: function and diversity A.R. Robart, S. Zimmerly Department of Biological Sciences, University of Calgary, Calgary, Alberta (Canada) Manuscript received 16 October 2003; accepted in revised form for publication by J.-N. Volff 8 December 2003. Abstract. Group II introns are a class of retroelements capa- and bacterial introns with regard to structures, insertion pat- ble of carrying out both self-splicing and retromobility reac- terns and inferred behaviors. We also discuss the evolution of tions. In recent years, the number of known group II introns has group II introns, as they are the putative ancestors of spliceoso- increased dramatically, particularly in bacteria, and the new mal introns and possibly non-LTR retroelements, and may information is altering our understanding of these intriguing have played an important role in the development of eukaryote elements. Here we review the basic properties of group II genomes. introns, and summarize the differences between the organellar Copyright © 2005 S. Karger AG, Basel Group II introns are a unique type of genetic element with catalytic core of the ribozyme (Fig. 1A) (Michel and Ferat, two remarkable properties. They are catalytic RNAs, or ribo- 1995; Qin and Pyle, 1998). Domain I is the largest domain and zymes, that can excise themselves from pre-mRNA without the is also important for catalysis, while domain VI contains the aid of proteins. They are also retroelements that encode reverse bulged A that forms the branch site in the spliced product. -
Homeostasis Is Critical for Group II Intron Splicing in Vivo
Downloaded from genesdev.cshlp.org on September 24, 2021 - Published by Cold Spring Harbor Laboratory Press Mitochondrial Mg2+ homeostasis is critical for group II intron splicing in vivo Juraj Gregan,1 Martin Kolisek, and Rudolf J. Schweyen2 Vienna Biocenter, Department of Microbiology and Genetics, University of Vienna, A-1030 Vienna, Austria The product of the nuclear MRS2 gene, Mrs2p, is the only candidate splicing factor essential for all group II introns in mitochondria of the yeast Saccharomyces cerevisiae. It has been shown to be an integral protein of the inner mitochondrial membrane, structurally and functionally related to the bacterial CorA Mg2+ transporter. Here we show that mutant alleles of the MRS2 gene as well as overexpression of this gene both increase intramitochondrial Mg2+ concentrations and compensate for splicing defects of group II introns in mit− mutants M1301 and B-loop. Yet, covariation of Mg2+ concentrations and splicing is similarly seen when some other genes affecting mitochondrial Mg2+ concentrations are overexpressed in an mrs2⌬ mutant, indicating that not the Mrs2 protein per se but certain Mg2+ concentrations are essential for group II intron splicing. This critical role of Mg2+ concentrations for splicing is further documented by our observation that pre-mRNAs, accumulated in mitochondria isolated from mutants, efficiently undergo splicing in organello when these mitochondria are incubated in the presence of 10 mM external Mg2+ (mit− M1301) and an ionophore (mrs2⌬). This finding of an exceptional sensitivity of group II intron splicing toward Mg2+ concentrations in vivo is unprecedented and raises the question of the role of Mg2+ in other RNA-catalyzed reactions in vivo. -
The Rise and Falls of Introns
Heredity (2006) 96, 208–213 & 2006 Nature Publishing Group All rights reserved 0018-067X/06 $30.00 www.nature.com/hdy REVIEW The rise and falls of introns R Belshaw1 and D Bensasson2 1Department of Zoology, University of Oxford, South Parks Road, Oxford OX1 3PS, UK; 2Department of Biological Sciences, Imperial College London, Silwood Park Campus, Ascot SL5 7PY, UK There has been a lively debate over the evolution of quent gain and loss of introns appears to be an ongoing eukaryote introns: at what point in the tree of life did they process in many organisms. Some introns are now function- appear and from where, and what has been their subsequent ally important and there have been suggestions that invoke pattern of loss and gain? A diverse range of recent research natural selection for the ancient and recent gain of introns, papers is relevant to this debate, and it is timely to bring them but it is also possible that fixation and loss of introns can together. The absence of introns that are not self-splicing in occur in the absence of positive selection. prokaryotes and several other lines of evidence suggest an Heredity (2006) 96, 208–213. doi:10.1038/sj.hdy.6800791; ancient eukaryotic origin for these introns, and the subse- published online 1 February 2006 Keywords: group II intron; selfish DNA; effective population size; introns early; introns late; spliceosome Introduction are intron movements now, and are there unifying explanations for intron diversity in the different lineages Nuclear genes of eukaryotes typically contain multiple of life? Many recent studies are highly pertinent to these regions called introns that are removed from the pre- questions, and we review them briefly below. -
An Organellar Maturase Associates with Multiple Group II Introns
An organellar maturase associates with multiple group II introns Reimo Zoschkea, Masayuki Nakamurab, Karsten Lierea, Masahiro Sugiurab, Thomas Börnera, and Christian Schmitz-Linnewebera,1 aInstitute of Biology, Humboldt University,10115 Berlin, Germany; and bGraduate School of Natural Sciences, Nagoya City University, Nagoya 467-8501, Japan Edited by Alan M. Lambowitz, The University of Texas at Austin, Austin, TX, and approved December 18, 2009 (received for review August 19, 2009) Bacterial group II introns encode maturase proteins required for gesting that a chloroplast reading frame is required for splicing splicing. In organelles of photosynthetic land plants, most of the these multiple introns (7, 15, 16). As there are no other candi- group II introns have lost the reading frames for maturases. Here, dates for RNA processing factors in the well-described and small we show that the plastidial maturase MatK not only interacts with chloroplast genome, it was suggested that matK is responsible for its encoding intron within trnK-UUU, but also with six additional splicing these introns. If true, MatK would be an example of a group II introns, all belonging to intron subclass IIA. Mapping anal- group II intron maturase that has left the strict association with yses of RNA binding sites revealed MatK to recognize multiple just a single target intron, joined by the group I intron maturase regions within the trnK intron. Organellar group II introns are BI4 from yeast mitochondria, which supports splicing of two considered to be the ancestors of nuclear spliceosomal introns. group I introns (17). That MatK associates with multiple intron ligands makes it an Attempts to generate knock-out alleles of matK to more attractive model for an early trans-acting nuclear splicing activity.