Possible Role of Wetlands, Permafrost, and Methane Hydrates in the Methane Cycle Under Future Climate Change: a Review F
Total Page:16
File Type:pdf, Size:1020Kb
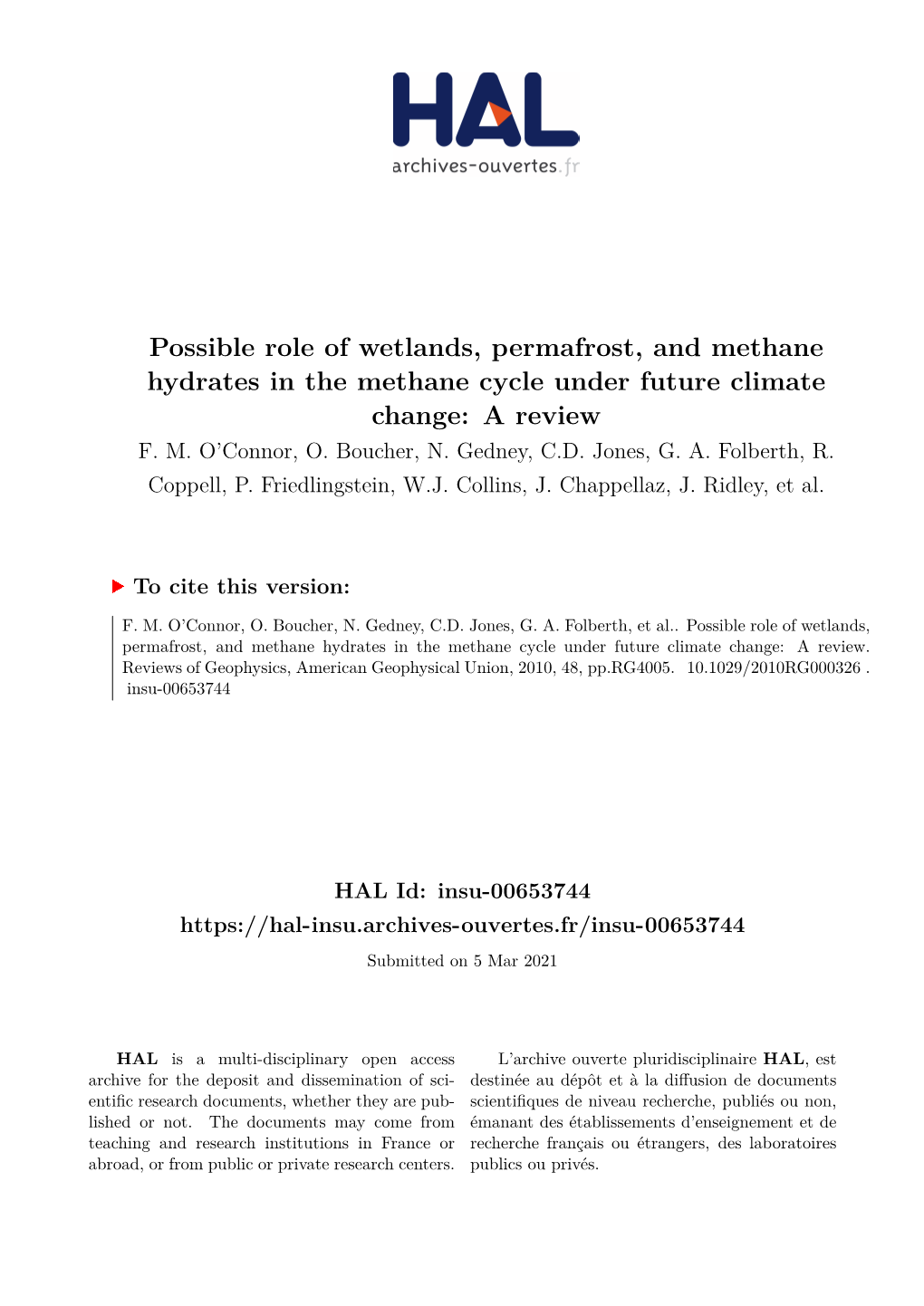
Load more
Recommended publications
-
Surficial Geology Investigations in Wellesley Basin and Nisling Range, Southwest Yukon J.D
Surficial geology investigations in Wellesley basin and Nisling Range, southwest Yukon J.D. Bond, P.S. Lipovsky and P. von Gaza Surficial geology investigations in Wellesley basin and Nisling Range, southwest Yukon Jeffrey D. Bond1 and Panya S. Lipovsky2 Yukon Geological Survey Peter von Gaza3 Geomatics Yukon Bond, J.D., Lipovsky, P.S. and von Gaza, P., 2008. Surficial geology investigations in Wellesley basin and Nisling Range, southwest Yukon. In: Yukon Exploration and Geology 2007, D.S. Emond, L.R. Blackburn, R.P. Hill and L.H. Weston (eds.), Yukon Geological Survey, p. 125-138. ABSTRACT Results of surficial geology investigations in Wellesley basin and the Nisling Range can be summarized into four main highlights, which have implications for exploration, development and infrastructure in the region: 1) in contrast to previous glacial-limit mapping for the St. Elias Mountains lobe, no evidence for the late Pliocene/early Pleistocene pre-Reid glacial limits was found in the study area; 2) placer potential was identified along the Reid glacial limit where a significant drainage diversion occurred for Grayling Creek; 3) widespread permafrost was encountered in the study area including near-continuous veneers of sheet-wash; and 4) a monitoring program was initiated at a recently active landslide which has potential to develop into a catastrophic failure that could damage the White River bridge on the Alaska Highway. RÉSUMÉ Les résultats d’études géologiques des formations superficielles dans le bassin de Wellesley et la chaîne Nisling peuvent être résumés en quatre principaux faits saillants qui ont des répercussions pour l’exploration, la mise en valeur et l’infrastructure de la région. -
The Main Features of Permafrost in the Laptev Sea Region, Russia – a Review
Permafrost, Phillips, Springman & Arenson (eds) © 2003 Swets & Zeitlinger, Lisse, ISBN 90 5809 582 7 The main features of permafrost in the Laptev Sea region, Russia – a review H.-W. Hubberten Alfred Wegener Institute for Polar and marine Research, Potsdam, Germany N.N. Romanovskii Geocryological Department, Faculty of Geology, Moscow State University, Moscow, Russia ABSTRACT: In this paper the concepts of permafrost conditions in the Laptev Sea region are presented with spe- cial attention to the following results: It was shown, that ice-bearing and ice-bonded permafrost exists presently within the coastal lowlands and under the shallow shelf. Open taliks can develop from modern and palaeo river taliks in active fault zones and from lake taliks over fault zones or lithospheric blocks with a higher geothermal heat flux. Ice-bearing and ice-bonded permafrost, as well as the zone of gas hydrate stability, form an impermeable regional shield for groundwater and gases occurring under permafrost. Emission of these gases and discharge of ground- water are possible only in rare open taliks, predominantly controlled by fault tectonics. Ice-bearing and ice-bonded permafrost, as well as the zone of gas hydrate stability in the northern region of the lowlands and in the inner shelf zone, have preserved during at least four Pleistocene climatic and glacio-eustatic cycles. Presently, they are subject to degradation from the bottom under the impact of the geothermal heat flux. 1 INTRODUCTION extremely complex. It consists of tectonic structures of different ages, including several rift zones (Tectonic This paper summarises the results of the studies of Map of Kara and Laptev Sea, 1998; Drachev et al., both offshore and terrestrial permafrost in the Laptev 1999). -
Chapter 7 Seasonal Snow Cover, Ice and Permafrost
I Chapter 7 Seasonal snow cover, ice and permafrost Co-Chairmen: R.B. Street, Canada P.I. Melnikov, USSR Expert contributors: D. Riseborough (Canada); O. Anisimov (USSR); Cheng Guodong (China); V.J. Lunardini (USA); M. Gavrilova (USSR); E.A. Köster (The Netherlands); R.M. Koerner (Canada); M.F. Meier (USA); M. Smith (Canada); H. Baker (Canada); N.A. Grave (USSR); CM. Clapperton (UK); M. Brugman (Canada); S.M. Hodge (USA); L. Menchaca (Mexico); A.S. Judge (Canada); P.G. Quilty (Australia); R.Hansson (Norway); J.A. Heginbottom (Canada); H. Keys (New Zealand); D.A. Etkin (Canada); F.E. Nelson (USA); D.M. Barnett (Canada); B. Fitzharris (New Zealand); I.M. Whillans (USA); A.A. Velichko (USSR); R. Haugen (USA); F. Sayles (USA); Contents 1 Introduction 7-1 2 Environmental impacts 7-2 2.1 Seasonal snow cover 7-2 2.2 Ice sheets and glaciers 7-4 2.3 Permafrost 7-7 2.3.1 Nature, extent and stability of permafrost 7-7 2.3.2 Responses of permafrost to climatic changes 7-10 2.3.2.1 Changes in permafrost distribution 7-12 2.3.2.2 Implications of permafrost degradation 7-14 2.3.3 Gas hydrates and methane 7-15 2.4 Seasonally frozen ground 7-16 3 Socioeconomic consequences 7-16 3.1 Seasonal snow cover 7-16 3.2 Glaciers and ice sheets 7-17 3.3 Permafrost 7-18 3.4 Seasonally frozen ground 7-22 4 Future deliberations 7-22 Tables Table 7.1 Relative extent of terrestrial areas of seasonal snow cover, ice and permafrost (after Washburn, 1980a and Rott, 1983) 7-2 Table 7.2 Characteristics of the Greenland and Antarctic ice sheets (based on Oerlemans and van der Veen, 1984) 7-5 Table 7.3 Effect of terrestrial ice sheets on sea-level, adapted from Workshop on Glaciers, Ice Sheets and Sea Level: Effect of a COylnduced Climatic Change. -
The Effects of Permafrost Thaw on Soil Hydrologic9 Thermal, and Carbon
Ecosystems (2012) 15: 213-229 DO!: 10.1007/sl0021-0!l-9504-0 !ECOSYSTEMS\ © 2011 Springer Science+Business Media, LLC (outside the USA) The Effects of Permafrost Thaw on Soil Hydrologic9 Thermal, and Carbon Dynan1ics in an Alaskan Peatland Jonathan A. O'Donnell,1 * M. Torre Jorgenson, 2 Jennifer W. Harden, 3 A. David McGuire,4 Mikhail Z. Kanevskiy, 5 and Kimberly P. Wickland1 1 U.S. Geological Survey, 3215 Marine St., Suite E-127, Boulder, Colorado 80303, USA; 2 Alaska Ecoscience, Fairbanks, Alaska 99775, USA; 3 U.S. Geological Survey, 325 Middlefield Rd, MS 962, Menlo Park, California 94025, USA; 4 U.S. Geological Survey, Alaska Cooperative Fish and Wildlife Research Unit, Institute of Arctic Biology, University of Alaska Fairbanks, Fairbanks, Alaska 99775, USA; 5/nstitute of Northern Engineering, University of Alaska Fairbanks, Fairbanks, Alaska 99775, USA ABSTRACT Recent warming at high-latitudes has accelerated tion of thawed forest peat reduced deep OC stocks by permafrost thaw in northern peatlands, and thaw can nearly half during the first 100 years following thaw. have profound effects on local hydrology and eco Using a simple mass-balance model, we show that system carbon balance. To assess the impact of per accumulation rates at the bog surface were not suf mafrost thaw on soil organic carbon (OC) dynamics, ficient to balance deep OC losses, resulting in a net loss we measured soil hydrologic and thermal dynamics of OC from the entire peat column. An uncertainty and soil OC stocks across a collapse-scar bog chrono analysis also revealed that the magnitude and timing sequence in interior Alaska. -
East Siberian Arctic Inland Waters Emit Mostly Contemporary Carbon ✉ Joshua F
ARTICLE https://doi.org/10.1038/s41467-020-15511-6 OPEN East Siberian Arctic inland waters emit mostly contemporary carbon ✉ Joshua F. Dean 1,2 , Ove H. Meisel1, Melanie Martyn Rosco1, Luca Belelli Marchesini 3,4, Mark H. Garnett 5, Henk Lenderink1, Richard van Logtestijn 6, Alberto V. Borges7, Steven Bouillon 8, Thibault Lambert7, Thomas Röckmann 9, Trofim Maximov10,11, Roman Petrov 10,11, Sergei Karsanaev10,11, Rien Aerts6, Jacobus van Huissteden1, Jorien E. Vonk1 & A. Johannes Dolman 1 1234567890():,; Inland waters (rivers, lakes and ponds) are important conduits for the emission of terrestrial carbon in Arctic permafrost landscapes. These emissions are driven by turnover of con- temporary terrestrial carbon and additional pre-aged (Holocene and late-Pleistocene) carbon released from thawing permafrost soils, but the magnitude of these source contributions to total inland water carbon fluxes remains unknown. Here we present unique simultaneous radiocarbon age measurements of inland water CO2,CH4 and dissolved and particulate organic carbon in northeast Siberia during summer. We show that >80% of total inland water carbon was contemporary in age, but pre-aged carbon contributed >50% at sites strongly affected by permafrost thaw. CO2 and CH4 were younger than dissolved and particulate organic carbon, suggesting emissions were primarily fuelled by contemporary carbon decomposition. Our findings reveal that inland water carbon emissions from permafrost landscapes may be more sensitive to changes in contemporary carbon turnover than the release of pre-aged carbon from thawing permafrost. 1 Department of Earth Sciences, Vrije Universiteit Amsterdam, Amsterdam, the Netherlands. 2 School of Environmental Sciences, University of Liverpool, Liverpool, UK. -
Mineral Element Stocks in the Yedoma Domain: a First Assessment in Ice-Rich Permafrost Regions” by Arthur Monhonval Et Al
Interactive comment on “Mineral element stocks in the Yedoma domain: a first assessment in ice-rich permafrost regions” by Arthur Monhonval et al. Anonymous Referee #2 Received and published: 4 January 2021 RC= Reviewer comment ; AR= Authors response RC: I appreciate the efforts from the authors. I understand the authors created a valuable dataset for the mineral elements in the yedoma regions, and they also tried to calculated the storage of these elements. I have some comments for the authors to improve the quality of the manuscripts. We thank the reviewer for the valuable comments and suggestions to improve the manuscript. We have revised the manuscript accordingly. Please find the details in the responses to the following comments. RC1: When the authors introduce the stocks or storage, it is necessary to clarify the depth or thickness of yedoma. At least, the authors should explain the characteristics of yedoma. This is important because the potential readers will be confused about the depth and height in the dataset. AR : We agree that the choice of the thickness used to upscale to the whole Yedoma domain was not clear in the manuscript. Here, mineral element stocks are compared with C stocks using identical Yedoma domain deposits parameters (including thicknesses) like in Strauss et al., 2013 for deep permafrost carbon pool of the Yedoma region, i.e., a mean thickness of 19.6 meters deep in Yedoma deposits and 5.5 meters deep in Alas deposits. We have revised the manuscript to include that information (L 282):” Thickness used for mineral element stock estimations in Yedoma domain deposits are based on mean profile depths of the sampled Yedoma (n=19) and Alas (n=10) deposits (Table 3; Strauss et al., 2013). -
Permafrost Soils and Carbon Cycling
SOIL, 1, 147–171, 2015 www.soil-journal.net/1/147/2015/ doi:10.5194/soil-1-147-2015 SOIL © Author(s) 2015. CC Attribution 3.0 License. Permafrost soils and carbon cycling C. L. Ping1, J. D. Jastrow2, M. T. Jorgenson3, G. J. Michaelson1, and Y. L. Shur4 1Agricultural and Forestry Experiment Station, Palmer Research Center, University of Alaska Fairbanks, 1509 South Georgeson Road, Palmer, AK 99645, USA 2Biosciences Division, Argonne National Laboratory, Argonne, IL 60439, USA 3Alaska Ecoscience, Fairbanks, AK 99775, USA 4Department of Civil and Environmental Engineering, University of Alaska Fairbanks, Fairbanks, AK 99775, USA Correspondence to: C. L. Ping ([email protected]) Received: 4 October 2014 – Published in SOIL Discuss.: 30 October 2014 Revised: – – Accepted: 24 December 2014 – Published: 5 February 2015 Abstract. Knowledge of soils in the permafrost region has advanced immensely in recent decades, despite the remoteness and inaccessibility of most of the region and the sampling limitations posed by the severe environ- ment. These efforts significantly increased estimates of the amount of organic carbon stored in permafrost-region soils and improved understanding of how pedogenic processes unique to permafrost environments built enor- mous organic carbon stocks during the Quaternary. This knowledge has also called attention to the importance of permafrost-affected soils to the global carbon cycle and the potential vulnerability of the region’s soil or- ganic carbon (SOC) stocks to changing climatic conditions. In this review, we briefly introduce the permafrost characteristics, ice structures, and cryopedogenic processes that shape the development of permafrost-affected soils, and discuss their effects on soil structures and on organic matter distributions within the soil profile. -
Dissolved Organic Carbon (DOC) in Arctic Ground
Discussion Paper | Discussion Paper | Discussion Paper | Discussion Paper | The Cryosphere Discuss., 9, 77–114, 2015 www.the-cryosphere-discuss.net/9/77/2015/ doi:10.5194/tcd-9-77-2015 © Author(s) 2015. CC Attribution 3.0 License. This discussion paper is/has been under review for the journal The Cryosphere (TC). Please refer to the corresponding final paper in TC if available. Dissolved organic carbon (DOC) in Arctic ground ice M. Fritz1, T. Opel1, G. Tanski1, U. Herzschuh1,2, H. Meyer1, A. Eulenburg1, and H. Lantuit1,2 1Alfred Wegener Institute, Helmholtz Centre for Polar and Marine Research, Department of Periglacial Research, Telegrafenberg A43, 14473 Potsdam, Germany 2Potsdam University, Institute of Earth and Environmental Sciences, Karl-Liebknecht-Str. 24–25, 14476 Potsdam-Golm, Germany Received: 5 November 2014 – Accepted: 13 December 2014 – Published: 7 January 2015 Correspondence to: M. Fritz ([email protected]) Published by Copernicus Publications on behalf of the European Geosciences Union. 77 Discussion Paper | Discussion Paper | Discussion Paper | Discussion Paper | Abstract Thermal permafrost degradation and coastal erosion in the Arctic remobilize substan- tial amounts of organic carbon (OC) and nutrients which have been accumulated in late Pleistocene and Holocene unconsolidated deposits. Their vulnerability to thaw 5 subsidence, collapsing coastlines and irreversible landscape change is largely due to the presence of large amounts of massive ground ice such as ice wedges. However, ground ice has not, until now, been considered to be a source of dissolved organic carbon (DOC), dissolved inorganic carbon (DIC) and other elements, which are impor- tant for ecosystems and carbon cycling. Here we show, using geochemical data from 10 a large number of different ice bodies throughout the Arctic, that ice wedges have the 1 1 greatest potential for DOC storage with a maximum of 28.6 mg L− (mean: 9.6 mg L− ). -
Spatial and Temporal Dynamics of Microorganisms Living Along Steep Energy Gradients and Implications for Ecology and Geologic Preservation in the Deep Biosphere
Spatial and Temporal Dynamics of Microorganisms Living Along Steep Energy Gradients and Implications for Ecology and Geologic Preservation in the Deep Biosphere Thesis by Sean William Alexander Mullin In Partial Fulfillment of the Requirements for the degree of Doctor of Philosophy CALIFORNIA INSTITUTE OF TECHNOLOGY Pasadena, California 2020 (Defended 8 June 2020) ii ã 2020 Sean W. A. Mullin ORCID: 0000-0002-6225-3279 iii What is any man’s discourse to me, if I am not sensible of something in it as steady and cheery as the creak of crickets? In it the woods must be relieved against the sky. Men tire me when I am not constantly greeted and refreshed as by the flux of sparkling streams. Surely joy is the condition of life. Think of the young fry that leap in ponds, the myriads of insects ushered into being on a summer evening, the incessant note of the hyla with which the woods ring in the spring, the nonchalance of the butterfly carrying accident and change painted in a thousand hues upon its wings… —Henry David Thoreau, “Natural History of Massachusetts” iv ACKNOWLEDGEMENTS Seven years is a long time. Beyond four years, the collective memory of a university is misty and gray, and if it were a medieval map, would be marked simply, “Here be dragons.” The number of times I have been mistaken this past year for an aged staff scientist or long-suffering post-doc would be amusing if not for my deepening wrinkles serving to confirm my status as a relative dinosaur. Wrinkles aside, I can happily say that my time spent in the Orphan Lab has been one of tremendous growth and exploration. -
Supplement of Observation-Based Modelling of Permafrost Carbon fluxes with Accounting for Deep Carbon Deposits and Thermokarst Activity
Supplement of Biogeosciences, 12, 3469–3488, 2015 http://www.biogeosciences.net/12/3469/2015/ doi:10.5194/bg-12-3469-2015-supplement © Author(s) 2015. CC Attribution 3.0 License. Supplement of Observation-based modelling of permafrost carbon fluxes with accounting for deep carbon deposits and thermokarst activity T. Schneider von Deimling et al. Correspondence to: T. Schneider von Deimling ([email protected]) The copyright of individual parts of the supplement might differ from the CC-BY 3.0 licence. 1. Terminology and definitions Active layer: The layer of ground that is subject to annual thawing and freezing in areas underlain by permafrost (van Everdingen, 2005) Differentiation between Mineral Soils and Organic Soils: Mineral soils: Including mineral soil material (less than 2.0 mm in diameter) and contain less than 20 percent (by weight) organic carbon (Soil Survey Staff, 1999). Here including Orthels and Turbels (see definition below). Organic soils: Soils including a large amount of organic carbon (more than 20 percent by weight, Soil Survey Staff, 1999). Here including Histels (see definition below). Gelisols: Soil order in the Soil taxonomy (Soil Survey Staff, 1999). Gelisols include soils of very cold climates, containing permafrost within the first 2m below surface. Gelisols are divided into 3 suborders: Histels: Including large amounts of organic carbon that commonly accumulate under anaerobic conditions (>80 vol % organic carbon from the soil surface to a depth of 50 cm) (Soil Survey Staff, 1999). Turbels: Gelisols that have one or more horizons with evidence of cryoturbation in the form of irregular, broken, or distorted horizon boundaries, involutions, the accumulation of organic matter on top of the permafrost, ice or sand wedges (Soil Survey Staff, 1999). -
A Differential Frost Heave Model: Cryoturbation-Vegetation Interactions
A Differential Frost Heave Model: Cryoturbation-Vegetation Interactions R. A. Peterson, D. A. Walker, V. E. Romanovsky, J. A. Knudson, M. K. Raynolds University of Alaska Fairbanks, AK 99775, USA W. B. Krantz University of Cincinnati, OH 45221, USA ABSTRACT: We used field observations of frost-boil distribution, soils, and vegetation to attempt to vali- date the predictions of a Differential Frost Heave (DFH) model along the temperature gradient in northern Alaska. The model successfully predicts order of magnitude heave and spacing of frost boils, and can account for the circular motion of soils within frost boils. Modification of the model is needed to account for the ob- served variation in frost boil systems along the climate gradient that appear to be the result of complex inter- actions between frost heave and vegetation. 1 INTRODUCTION anced by degrading geological processes such as soil creep. Washburn listed 19 possible mechanisms re- This paper discusses a model for the development sponsible for the formation of patterned ground of frost boils due to differential frost heave. The in- (Washburn 1956). The model presented here ex- teractions between the physical processes of frost plains the formation of patterned ground by differen- heave and vegetation characteristics are explored as tial frost heave. DFH is also responsible, at least in a possible controlling mechanism for the occurrence part, for the formation of sorted stone circles in of frost boils on the Alaskan Arctic Slope. Spitsbergen (Hallet et al 1988). A recent model due to Kessler et al (2001) for the genesis and mainte- Frost boils are a type of nonsorted circle, “a pat- nance of stone circles integrates DFH with soil con- terned ground form that is equidimensional in sev- solidation, creep, and illuviation. -
Master's Thesis in Geography Physical Geography Mesoscale Modelling of Periglacial Landforms in the Circumpolar Arctic Tommi K
Master’s thesis in Geography Physical Geography Mesoscale modelling of periglacial landforms in the circumpolar Arctic Tommi Kukkonen 2020 Supervisor: Miska Luoto Master’s Programme in Geography University of Helsinki Faculty of Science Department of geosciences and geography P. O. Box 64 (Gustaf Hällströmin katu 2) 00014 University of Helsinki Tiedekunta – Fakultet – Faculty Koulutusohjelma – Utbildningsprogram – Degree programme Faculty of Science Master's Programme in Geography Opintosuunta – Studieinrikting – Study track Physical Geography Tekijä – Författare – Author Tommi Kukkonen Työn nimi – Arbetets titel – Title Mesoscale modelling of periglacial landforms in the circumpolar Arctic Työn laji – Arbetets art – Level Aika – Datum – Month and year Sivumäärä – Sidoantal – Number of pages Master's thesis May 2020 86 pages Tiivistelmä – Referat – Abstract The Arctic is warming with an increased pace, and it can affect ecosystems, infrastructure and communities. By studying periglacial landforms and processes, and using improved methods, more knowledge on these changing environmental conditions and their impacts can be obtained. The aim of this thesis is to map studied landforms and predict their probability of occurrence in the circumpolar region utilizing different modelling methods. Periglacial environments occur in high latitudes and other cold regions. These environments host permafrost, which is frozen ground and responds effectively to climate warming, and underlays areas that host many landform types. Therefore, landform monitoring and modelling in permafrost regions under changing climate can provide information about the ongoing changes in the Arctic and landform distributions. Here four landform/process types were mapped and studied: patterned ground, pingos, thermokarst activity and solifluction. The study consisted of 10 study areas across the circumpolar arctic that were mapped for their landforms.