U-232 and the Proliferation- Resistance of U-233 in Spent Fuel
Total Page:16
File Type:pdf, Size:1020Kb
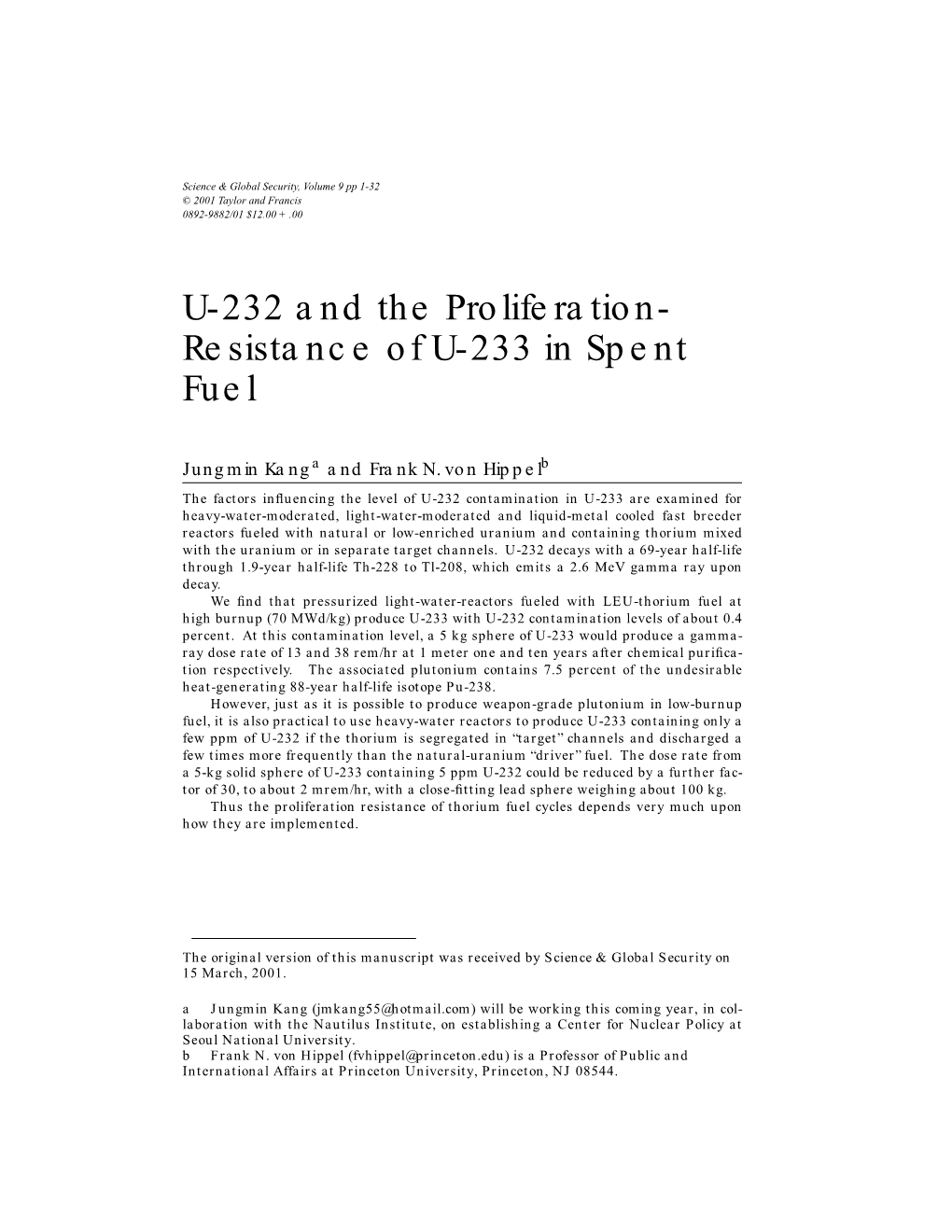
Load more
Recommended publications
-
Institute for Clinical and Economic Review
INSTITUTE FOR CLINICAL AND ECONOMIC REVIEW FINAL APPRAISAL DOCUMENT BRACHYTHERAPY & PROTON BEAM THERAPY FOR TREATMENT OF CLINICALLY-LOCALIZED, LOW-RISK PROSTATE CANCER December 22, 2008 Senior Staff Daniel A. Ollendorf, MPH, ARM Chief Review Officer Julia Hayes, MD Lead Decision Scientist Pamela McMahon, PhD Sr. Decision Scientist Steven D. Pearson, MD, MSc President, ICER Associate Staff Michelle Kuba, MPH Sr. Technology Analyst Angela Tramontano, MPH Research Assistant © ICER, 2008 1 CONTENTS About ICER .................................................................................................................................. 3 Acknowledgments ...................................................................................................................... 4 Executive Summary .................................................................................................................... 5 Evidence Review Group Deliberation.................................................................................. 15 ICER Integrated Evidence Rating.......................................................................................... 21 Evidence Review Group Members........................................................................................ 24 Appraisal Overview.................................................................................................................. 28 Background ............................................................................................................................... -
小型飛翔体/海外 [Format 2] Technical Catalog Category
小型飛翔体/海外 [Format 2] Technical Catalog Category Airborne contamination sensor Title Depth Evaluation of Entrained Products (DEEP) Proposed by Create Technologies Ltd & Costain Group PLC 1.DEEP is a sensor analysis software for analysing contamination. DEEP can distinguish between surface contamination and internal / absorbed contamination. The software measures contamination depth by analysing distortions in the gamma spectrum. The method can be applied to data gathered using any spectrometer. Because DEEP provides a means of discriminating surface contamination from other radiation sources, DEEP can be used to provide an estimate of surface contamination without physical sampling. DEEP is a real-time method which enables the user to generate a large number of rapid contamination assessments- this data is complementary to physical samples, providing a sound basis for extrapolation from point samples. It also helps identify anomalies enabling targeted sampling startegies. DEEP is compatible with small airborne spectrometer/ processor combinations, such as that proposed by the ARM-U project – please refer to the ARM-U proposal for more details of the air vehicle. Figure 1: DEEP system core components are small, light, low power and can be integrated via USB, serial or Ethernet interfaces. 小型飛翔体/海外 Figure 2: DEEP prototype software 2.Past experience (plants in Japan, overseas plant, applications in other industries, etc) Create technologies is a specialist R&D firm with a focus on imaging and sensing in the nuclear industry. Createc has developed and delivered several novel nuclear technologies, including the N-Visage gamma camera system. Costainis a leading UK construction and civil engineering firm with almost 150 years of history. -
A 50-100 Kwe Gas-Cooled Reactor for Use on Mars
SANDIA REPORT SAND2006-2189 Unlimited Release Printed April 2006 A 50-100 kWe Gas-cooled Reactor For Use On Mars Curtis D. Peters Prepared by Sandia National Laboratories Albuquerque, New Mexico 87185 and Livermore, California 94550 Sandia is a multiprogram laboratory operated by Sandia Corporation, a Lockheed Martin Company, for the United States Department of Energy’s National Nuclear Security Administration under Contract DE-AC04-94AL85000. Approved for public release; further dissemination unlimited. Issued by Sandia National Laboratories, operated for the United States Department of Energy by Sandia Corporation. NOTICE: This report was prepared as an account of work sponsored by an agency of the United States Government. Neither the United States Government, nor any agency thereof, nor any of their employees, nor any of their contractors, subcontractors, or their employees, make any warranty, express or implied, or assume any legal liability or responsibility for the accuracy, completeness, or usefulness of any information, apparatus, product, or process disclosed, or represent that its use would not infringe privately owned rights. Reference herein to any specific commercial product, process, or service by trade name, trademark, manufacturer, or otherwise, does not necessarily constitute or imply its endorsement, recommendation, or favoring by the United States Government, any agency thereof, or any of their contractors or subcontractors. The views and opinions expressed herein do not necessarily state or reflect those of the United States Government, any agency thereof, or any of their contractors. Printed in the United States of America. This report has been reproduced directly from the best available copy. Available to DOE and DOE contractors from U.S. -
Advances in Small Modular Reactor Technology Developments
Advances in Small Modular Reactor Technology Developments Advances in Small Modular Reactor Technology Developments Technology in Small Modular Reactor Advances A Supplement to: IAEA Advanced Reactors Information System (ARIS) 2018 Edition For further information: Nuclear Power Technology Development Section (NPTDS) Division of Nuclear Power IAEA Department of Nuclear Energy International Atomic Energy Agency Vienna International Centre PO Box 100 1400 Vienna, Austria Telephone: +43 1 2600-0 Fax: +43 1 2600-7 Email: [email protected] Internet: http://www.iaea.org Printed by IAEA in Austria September 2018 18-02989E ADVANCES IN SMALL MODULAR REACTOR TECHNOLOGY DEVELOPMENTS 2018 Edition A Supplement to: IAEA Advanced Reactors Information System (ARIS) http://aris.iaea.org DISCLAIMER This is not an official IAEA publication. The material has not undergone an official review by the IAEA. The views expressed do not necessarily reflect those of the International Atomic Energy Agency or its Member States and remain the responsibility of the contributors. Although great care has been taken to maintain the accuracy of information contained in this publication, neither the IAEA nor its Member States assume any responsibility for consequences which may arise from its use. The use of particular designations of countries or territories does not imply any judgement by the publisher, the IAEA, as to the legal status of such countries or territories, of their authorities and institutions or of the delimitation of their boundaries. The mention of names of specific companies or products (whether or not indicated as registered) does not imply any intention to infringe proprietary rights, nor should it be construed as an endorsement or recommendation on the part of the IAEA. -
Self-Sustaining Thorium Boiling Water Reactors
Project No. 11-3023 Self-Sustaining Thorium Boiling Water Reactors Reactor Concepts Dr. Ehud Greenspan University of California-Berkeley In collaboraon with: Massachuses Instute of Technology University of Michigan Brookhaven Naonal Laboratory Brian Robinson, Federal POC Temitope Taiwo, Technical POC NEUP Project # 11-3023 September 2011 to December 2014 Self-sustaining thorium boiling water reactors Summary Report Ehud Greenspan, Phillip M. Gorman, Sandra Bogetic, Jeffrey E. Seifried, Guanheng Zhang, Christopher R. Varela, Massimiliano Fratoni and Jasmina L. Vujic UC Berkeley, [email protected] Thomas Downar, Andrew Hall, Andrew Ward, Michael Jarrett, Aaron Wysocki and Yunlin Xu University of Michigan, [email protected] Mujid Kazimi, Koroush Shirvan and Alexander Mieloszyk MIT, [email protected] Michael Todosow, Nicholas Brown and Lap Cheng BNL, [email protected] 28 February 2015 1 Table of Contents Overview Page Executive summary 3 1. Introduction 7 2. The RBWR cores 7 2.1 Hitachi RBWR cores 7 2.2 Independent evaluation of the Hitachi designs 14 2.3 The incentive for thorium-based RBWR 15 2.4 The thorium-based RBWR core designs 17 3. Development of computational methods for RBWR type cores 19 3.1 3-D core simulator 20 3.2 Monte-Carlo based coupled neutronics – TH – depletion analysis capability 21 3.3 Stability and safety analysis capability 21 3.4 Fuel performance analysis capability 22 3.5 Thermal hydraulic correlations for tight lattice high void cores 24 4. Feasibility of fuel-self-sustaining RBWR-Th cores 24 4.1 Study strategy 24 4.2 RBWR-Th core designs 26 4.3 RBWR-Th safety and stability 30 4.4 RBWR-Th fuel performance 31 5. -
Latest Fusion R&D Activities at INEST
1 Latest Fusion R&D Activities at INEST Presented by Yican Wu (Zhibin Chen on behalf) Contributed by FDS Team Institute of Nuclear Energy Safety Technology (INEST) Chinese Academy of Sciences www.fds.org.cn FDS: Frontier Development of Science 2 Institute of Nuclear Energy Safety Technology Chinese Academy of Sciences ∙ FDS Team Main Research Areas Fundamental research: • Neutron Physics Applied research: 1. Advanced Fission Energy LFR etc. 2. Fusion Energy FNT & Materials etc. Headquarter in Hefei, Anhui, China 3. Extended Nuclear Technology Applications Key Programs: . Strategic Priority Research Program of CAS . ITER Related International and Domestic Program . Nuclear Energy & Safety Technology Innovation Program 400 Staff + 100 Students Three Integrated Test Reactors A professional institute is devoted to design and R&D of advanced nuclear energy and safety technology 3 Outline I. Highlights of Fusion R&D Activities • Fusion Neutron Sources • Neutronics Methodology and Simulation • Fusion Safety • Materials and TBM Technologies II. International Communication and Collaboration 4 1. Fusion Neutron Sources 5 Development of Fusion Neutron Sources at INEST ≥ 1018 n/ s 15 16 10 -10 n/s HINEG-IIIa 1012-1013 n/s Fusion Volumetric Neutron HINEG-IIa Source ( e.g. GDT-FVNS ) Mixed-beam HINEG-I Fusion Neutron Source (in Operation) . Fusion materials (≥20dpa/FPY) . Materials Irradiation . Multi-Physics Coupling Test . Nuclear Design and Performance . Nuclear Data . System Engineering Integration Test of Component Test . Methods and Software -
Control Rod Safety Management in Nuclear Power Plant
Journal of Advanced Review on Scientific Research 32, Issue 1 (2017) 14-27 Journal of Advanced Review on Scientific Penerbit Akademia Baru Research Journal homepage: www.akademiabaru.com/arsr.html ISSN: 2289-7887 Control rod safety m anage ment in nuclear power plant: A Open review Access Muhammad Adil Khattak 1, ∗, Filzah Hazirah Jaffar 1,Najaa Fadhilah Mohd Nasir 1, Nurfarhah 1 1 1 Ridzuan , Nurlaila Syamsul Bahri ,Wan Nur Iffah Haziqah Md Zamri 1 Department of Nuclear Engineering, Faculty of Chemical & Energy Engineering Universiti Teknologi Malaysia, 81310 Skudai Johor , Malaysia ARTICLE INFO ABSTRACT Article history: The primary objective of control rod management is to ensure the safe, reliable and Received 12 April 2017 optimum use of the nuclear fuel in the reactor, while remain within the limits imposed Received in revised form 24 May 2017 by the design of the fuel assembly and reactor w.r.t the safety analysis. In numerous Accepted 25 May 2017 reactors, the control rods perform the function of reactivity control, both globally and Available online 26 May 2017 locally, latter also control the power distribution of the core. Most control rods are completely withdrawn from the core during operations and fully inserted during shutdown of the reactor. This are the prove of an investigative study into optimization of the heterogeneous control rods, the safety management of an additional safety rod, and control rod drop hydrodynamic analysis which will be able to provide an efficient and maximum safety procedure for emergency shut down system in the reactor. It is also will be very important safety features of the reactor. -
NRC Collection of Abbreviations
I Nuclear Regulatory Commission c ElLc LI El LIL El, EEELIILE El ClV. El El, El1 ....... I -4 PI AVAILABILITY NOTICE Availability of Reference Materials Cited in NRC Publications Most documents cited in NRC publications will be available from one of the following sources: 1. The NRC Public Document Room, 2120 L Street, NW., Lower Level, Washington, DC 20555-0001 2. The Superintendent of Documents, U.S. Government Printing Office, P. 0. Box 37082, Washington, DC 20402-9328 3. The National Technical Information Service, Springfield, VA 22161-0002 Although the listing that follows represents the majority of documents cited in NRC publica- tions, it is not intended to be exhaustive. Referenced documents available for inspection and copying for a fee from the NRC Public Document Room include NRC correspondence and internal NRC memoranda; NRC bulletins, circulars, information notices, inspection and investigation notices; licensee event reports; vendor reports and correspondence; Commission papers; and applicant and licensee docu- ments and correspondence. The following documents in the NUREG series are available for purchase from the Government Printing Office: formal NRC staff and contractor reports, NRC-sponsored conference pro- ceedings, international agreement reports, grantee reports, and NRC booklets and bro- chures. Also available are regulatory guides, NRC regulations in the Code of Federal Regula- tions, and Nuclear Regulatory Commission Issuances. Documents available from the National Technical Information Service Include NUREG-series reports and technical reports prepared by other Federal agencies and reports prepared by the Atomic Energy Commission, forerunner agency to the Nuclear Regulatory Commission. Documents available from public and special technical libraries include all open literature items, such as books, journal articles, and transactions. -
Irradiation Performance of Fast Reactor Fuels
Oktober 1967 KFK 662 EUR 3698 e Institut für Angewandte Reaktorphysik Institut für Material- und Festkörperforschung Irradiation Performance of Fast Reactor Fuels D. Geithoff, G. Karsten, K. Kummerer GESELLSCHAFT FUR KERNF( KARLSRUHE Als Manuskript vervielfältigt Für diesen Bericht behalten wir uns alle Rechte vor Gesellschaft für 'Kernforschung m.b.H. Karlsruhe KERNFORSCHUNGSZENTRUM KARLSRUHE Oktober I967 KFK662 EUR 3698 e Institut für Angewandte Reaktorphysik Institut für Material und Festkörperforschung IRRADIATION PERFORMANCE OF FAST REACTOR FUELS *' D. Geithoff, G. Karsten and Κ. Kümmerer Paper presented at the Symposium on Plutonium Fuels Technology, Nuclear Metallurgy Committee IMDAIME, Oct.46,1967, Phoenix, Ariz., USA. Gesellschaft für Kernforschung mbH., Karlsruhe *) Work performed within the association in the field of fast reactors between the European Atomic Energy Community and Gesellschaft für Kernforschung mbH., Karlsruhe ABSTRACT The requirements for uranium-plutonium dioxide fuels are the basis for a program of irradiation tests which is outlined in the introduction. Some hypothetical considerations supply a rational background for the design of specimens and the chosen operation conditions. The experimental facilities and irradiation devices are shortly described and discussed with respect to the irradiation characteristics. The available hot cell equipment provides for the standard examination technique including X-raying by betatron and fission gas chromatography. One group of experiments refers to the short term behaviour of the oxide type fuel. In another group the long time behaviour of oxide fuel pins is studied. Different pins have been irradiated in capsules and in a helium loop. The present results indicate, that the basic assumptions for the pin design are principally con firmed, the specimen failures only arising from malfunctions of the irradiation devices. -
Nuclear Navy United States Atomic Energy Commission Historical Advisory Committee
Nuclear Navy United States Atomic Energy Commission Historical Advisory Committee Chairman, Alfred D. Chandler, Jr. Harvard University John T. Conway Consolidated Edison Company Lauchlin M. Currie Carmel, California A. Hunter Dupree Brown University Ernest R. May Harvard University Robert P. Multhauf Smithsonian Institution Nuclear Navy 1946-1962 Richard G. Hewlett and Francis Duncan The University of Chicago Press Chicago and London The University of Chicago Press Chicago 60637 The University of Chicago Press Ltd., London Published 1974 Printed in the United States of America International Standard Book Number: 0-226-33219-5 Library of Congress Catalog Card Number: 74-5726 RICHARD G. HEWLETT is chief historian of the U. S. Atomic Energy Commission. He is coauthor, with Oscar E. Anderson, Jr., of The New World, 1939-1946 and, with Francis Duncan, of Atomic Shield, 1947-1952. FRANCIS DUNCAN is assistant historian of the U.S. Atomic Energy Commission. He is the coauthor of Atomic Shield. [1974] VA Contents Illustrations vii Foreword ix Preface xi 1 2 3 4 Control The The The of the Idea Question of Structure Sea and the Leadership of Responsi- 1 Challenge 52 bility 15 88 5 6 7 8 Emerging Prototypes Toward Nuclear Patterns of and a Nuclear Power Technical Submarines Fleet Beyond Management 153 194 the Navy 121 225 9 10 11 12 Propulsion Building Fleet The for the the Nuclear Operation Measure Fleet Fleet and of Accom- 258 297 Maintenance plishment 340 377 Appendix 1: Table of Organization Abbreviations 404 393 Notes 405 Appendix 2: Construction of the Sources 453 Nuclear Navy 399 Index 461 Appendix 3: Financial Data 402 V Illustrations Charts 8. -
Understanding Non-Hodgkin Lymphoma
Understanding Non-Hodgkin Lymphoma A Guide for Patients, Survivors, and Loved Ones July 2018 Lymphoma Research Foundation (LRF) Helpline and Clinical Trials Information Service CONTACT THE LRF HELPLINE Trained staff are available to answer questions and provide support to patients, caregivers and healthcare professionals in any language. Our support services include: • Information on lymphoma, treatment options, side effect management and current research fi ndings • Financial assistance for eligible patients and referrals for additional fi nancial, legal and insurance help • Clinical trial searches based on patient’s diagnosis and treatment history • Support through LRF’s Lymphoma Support Network, a national one-to one volunteer patient peer program Monday through Friday, Toll-Free (800) 500-9976 or email [email protected] Understanding Non-Hodgkin Lymphoma A Guide For Patients, Survivors, and Loved Ones July 2018 This guide is an educational resource compiled by the Lymphoma Research Foundation to provide general information on adult non- Hodgkin lymphoma. Publication of this information is not intended to replace individualized medical care or the advice of a patient’s doctor. Patients are strongly encouraged to talk to their doctors for complete information on how their disease should be diagnosed, treated, and followed. Before starting treatment, patients should discuss the potential benefits and side effects of cancer therapies with their physician. Contact the Lymphoma Research Foundation Helpline: (800) 500-9976 [email protected] Website: lymphoma.org This patient guide is supported through unrestricted educational grants from: © 2018 Lymphoma Research Foundation. Information contained herein is the property of the Lymphoma Research Foundation (LRF). Any portion may be reprinted or reproduced provided that LRF is acknowledged to be the source and the Foundation’s website (lymphoma.org) is included in the citation. -
Rickover and the Nuclear Navy Rickover and the Nuclear Navy
The Preface on pages xvii-xix has been altered to correct a typesetting error in the printed book. All of the text is unchanged, but sections of the text have been rearranged to place paragraphs in the proper order. Rickover and the Nuclear Navy Rickover and the Nuclear Navy THE DISCIPLINE OF TECHNOLOGY by Francis Duncan Naval Institute Press Annapolis, Maryland Published 1989 by the United States Naval Institute Annapolis, Maryland Copyright © 1989 on the foreword All rights reserved. Prepared by the Department of Energy; work made for hire. Library of Congress Cataloging-in-Publication Data Duncan, Francis, 1922- Rickover and the nuclear navy : the discipline of technology / by Francis Duncan. p. cm. Includes bibliographical references. ISBN 0-87021-236-2 1. Rickover, Hyman George. 2. Nuclear submarines—United States— History. 3. Admirals—United States—Biography 4. United States. Navy—Biography. I. Title. V63.R54D86 1989 359.3'2574'0973—dc20 89-39097 CIP This edition is authorized for sale only in the United States, its territories and possessions, and Canada. Printed in the United States of America 9 8 7 6 5 4 3 2 First printing Contents Foreword vii Preface xvii Acknowledgments xxv 1. Common Denominators 1 2. Submarines 17 3. Thresher 52 4. Surface Ships—First Battles 99 5. Surface Ships—The Alliance with Congress 115 6. Surface Ships—Legislating Nuclear Power into the Fleet 147 7. Technology and Diplomacy: The Multilateral Force 170 8. Shippingport 190 9. The Devil Is in the Details 232 10. Independence and Control 252 11. Discipline of Technology 279 Appendix 1.