Comparative Analysis of SN 2012Dn Optical Spectra: Days€−14 to +114
Total Page:16
File Type:pdf, Size:1020Kb
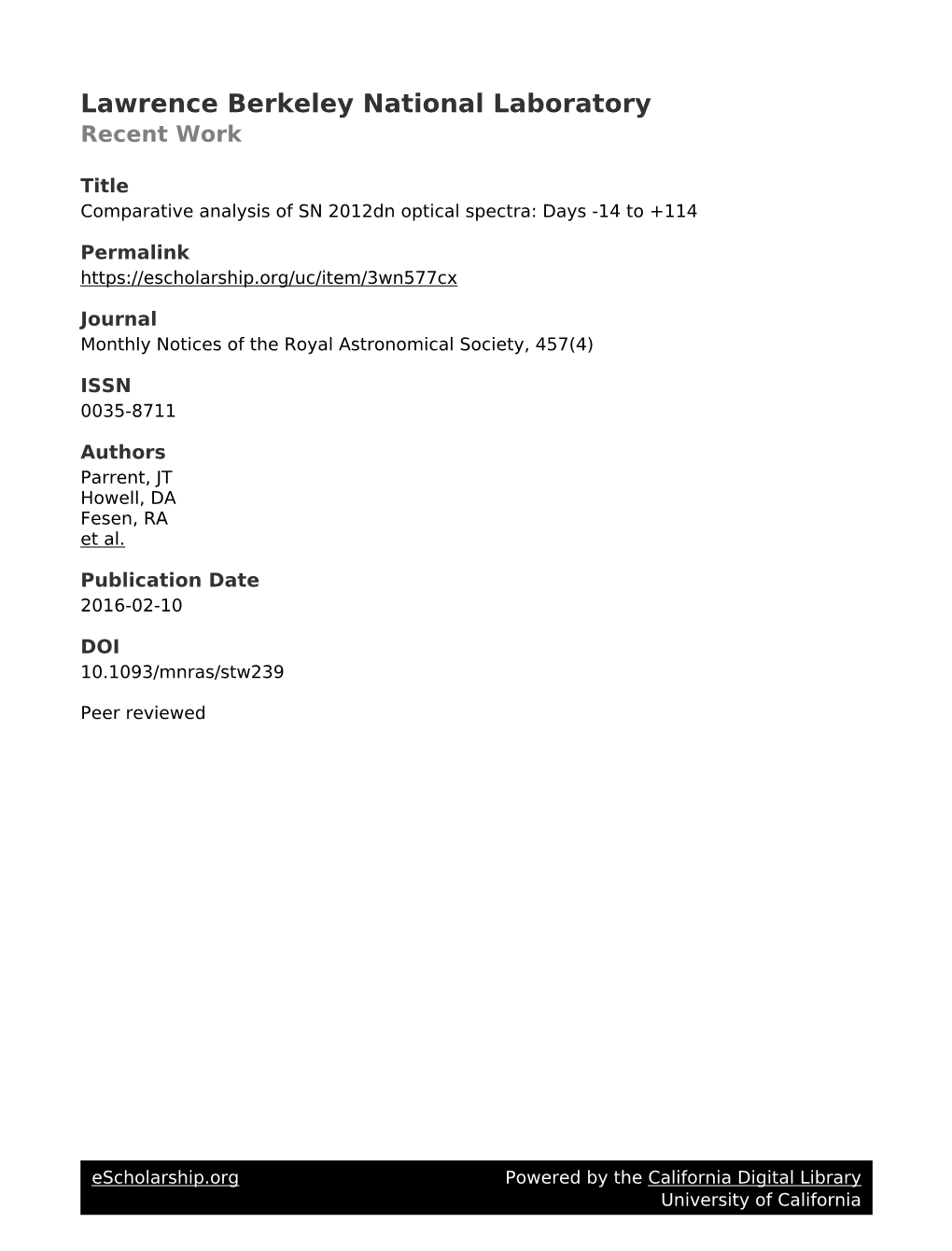
Load more
Recommended publications
-
LIKE SN 1991T OR SN 2007If? Ju-Jia Zhang1,2,3, Xiao-Feng Wang3, Michele Sasdelli4,5, Tian-Meng Zhang6, Zheng-Wei Liu7, Paolo A
The Astrophysical Journal, 817:114 (13pp), 2016 February 1 doi:10.3847/0004-637X/817/2/114 © 2016. The American Astronomical Society. All rights reserved. A LUMINOUS PECULIAR TYPE IA SUPERNOVA SN 2011HR: MORE LIKE SN 1991T OR SN 2007if? Ju-Jia Zhang1,2,3, Xiao-Feng Wang3, Michele Sasdelli4,5, Tian-Meng Zhang6, Zheng-Wei Liu7, Paolo A. Mazzali4,5, Xiang-Cun Meng1,2, Keiichi Maeda8,9, Jun-Cheng Chen3, Fang Huang3, Xu-Lin Zhao3, Kai-Cheng Zhang3, Qian Zhai1,2,10, Elena Pian11,12, Bo Wang1,2, Liang Chang1,2, Wei-Min Yi1,2, Chuan-Jun Wang1,2,10, Xue-Li Wang1,2, Yu-Xin Xin1,2, Jian-Guo Wang1,2, Bao-Li Lun1,2, Xiang-Ming Zheng1,2, Xi-Liang Zhang1,2, Yu-Feng Fan1,2, and Jin-Ming Bai1,2 1 Yunnan Observatories (YNAO), Chinese Academy of Sciences, Kunming 650011, China; [email protected] 2 Key Laboratory for the Structure and Evolution of Celestial Objects, Chinese Academy of Sciences, Kunming 650011, China 3 Physics Department and Tsinghua Center for Astrophysics (THCA), Tsinghua University, Beijing 100084, China; [email protected] 4 Astrophysics Research Institute, Liverpool John Moores University, Liverpool Science Park, 146 Brownlow Hill, Liverpool L3 5RF, UK 5 Max-Planck Institute fur Astrophysics, D-85748 Garching, Germany 6 National Astronomical Observatories of China (NAOC), Chinese Academy of Sciences, Beijing 100012, China 7 Argelander-Institut für Astronomie, Auf dem Hügel 71, D-53121, Bonn, Germany 8 Department of Astronomy, Kyoto University, Kyoto, 606-8502, Japan 9 Kavli Institute for the Physics and Mathematics of the Universe -
Highly Magnetized Super-Chandrasekhar White Dwarfs and Their Consequences
Highly magnetized super-Chandrasekhar white dwarfs and their consequences Banibrata Mukhopadhyay Department of Physics Indian Institute of Science Collaborators: Upasana Das (JILA, Colorado), Mukul Bhattacharya (Texas, Austin), Sathyawageeswar Subramanian (Cambridge), Tanayveer Singh Bhatia (IISc/ANU), Subroto Mukerjee (IISc), A. R. Rao (TIFR) Stars with a stable magnetic field: from pre-main sequence to compact remnants August 28 to September 1, 2017, Brno, Czech Republic The talk is based on the following papers MUKHOPADHYAY, A. R. Rao, T. S. Bhatia, MNRAS (press), 2017 MUKHOPADHYAY, A. R. Rao, JCAP, 05, 007, 2016 S. Subramanian, MUKHOPADHYAY, MNRAS, 454, 752, 2015 U. Das, MUKHOPADHYAY, IJMPD, 24, 1544026, 2015 U. Das, MUKHOPADHYAY, JCAP, 05, 045, 2015b U. Das, MUKHOPADHYAY, JCAP, 05, 015, 2015a U. Das, MUKHOPADHYAY, Phys. Rev. D, 91, 028302, 2015c U. Das, MUKHOPADHYAY, JCAP, 06, 050, 2014a U. Das, MUKHOPADHYAY, MPLA, 29, 1450035, 2014b M. V. Vishal, MUKHOPADHYAY, Phys. Rev. C, 89, 065804, 2014 U. Das, MUKHOPADHYAY, Phys. Rev. Lett., 110, 071102, 2013a U. Das, MUKHOPADHYAY, A. R. Rao, ApJLett., 767, 14, 2013 U. Das, MUKHOPADHYAY, IJMPD, 22, 1342004, 2013b U. Das, MUKHOPADHYAY, Phys. Rev. D, 86, 041001, 2012a U. Das, MUKHOPADHYAY, IJMPD, 21, 1242001, 2012b A. Kundu, MUKHOPADHYAY, MPLA, 27, 1250084, 2012 Flow-Chart of Evolution of our Idea Since last 5 years or so, we have initiated exploring highly magnetized super-Chandrasekhar white dwarfs (B-WDs), explaining peculiar type Ia supernovae: Over-luminous Many groups joined -
The Ejected Mass Distribution of Type Ia Supernovae: a Significant Rate of Non-Chandrasekhar-Mass Progenitors
View metadata, citation and similar papers at core.ac.uk brought to you by CORE provided by The Australian National University MNRAS 445, 2535–2544 (2014) doi:10.1093/mnras/stu1808 The ejected mass distribution of Type Ia supernovae: a significant rate of non-Chandrasekhar-mass progenitors R. A. Scalzo,1,2‹ A. J. Ruiter1,2,3 andS.A.Sim2,4 1Research School of Astronomy and Astrophysics, Australian National University, Canberra, ACT 2611, Australia 2ARC Centre of Excellence for All-Sky Astrophysics (CAASTRO), The Australian National University, Cotter Road, Weston Creek ACT 2611 Australia 3Max-Planck-Institut fur¨ Astrophysik, Karl-Schwarzschild-Str. 1, D-85741 Garching bei Munchen,¨ Germany 4Astrophysics Research Centre, School of Mathematics and Physics, Queen’s University Belfast, Belfast, BT7 1NN, UK Accepted 2014 September 1. Received 2014 August 21; in original form 2014 June 30 Downloaded from ABSTRACT The ejected mass distribution of Type Ia supernovae (SNe Ia) directly probes progenitor evolutionary history and explosion mechanisms, with implications for their use as cosmological probes. Although the Chandrasekhar mass is a natural mass scale for the explosion of white http://mnras.oxfordjournals.org/ dwarfs as SNe Ia, models allowing SNe Ia to explode at other masses have attracted much recent attention. Using an empirical relation between the ejected mass and the light-curve 56 width, we derive ejected masses Mej and Ni masses MNi for a sample of 337 SNe Ia with redshifts z<0.7 used in recent cosmological analyses. We use hierarchical Bayesian inference to reconstruct the joint Mej–MNi distribution, accounting for measurement errors. -
Type Ia Supernovae As Stellar Endpoints and Cosmological Tools
Type Ia Supernovae as Stellar Endpoints and Cosmological Tools D. Andrew Howell1;2 1Las Cumbres Observatory Global Telescope Network, 6740 Cortona Dr., Suite 102, Goleta, CA 93117 2Department of Physics, University of California, Santa Barbara, Broida Hall, Mail Code 9530, Santa Barbara, CA 93106-9530 Empirically, Type Ia supernovae are the most useful, precise, and mature tools for determin- ing astronomical distances. Acting as calibrated candles they revealed the presence of dark energy and are being used to measure its properties. However, the nature of the Type Ia explosion, and the progenitors involved, have remained elusive, even after seven decades of research. But now new large surveys are bringing about a paradigm shift — we can finally compare samples of hundreds of supernovae to isolate critical variables. As a result of this, and advances in modeling, breakthroughs in understanding all aspects of these supernovae are finally starting to happen. “Guest stars” (who could have imagined they were distant stellar explosions) have been sur- prising humans for at least 950 years, but probably far longer. They amazed and confounded the likes of Tycho, Kepler, and Galileo, to name a few. But it was not until the separation of these events into novae and supernovae by Baade and Zwicky that progress understanding them began in earnest1. This process of splitting a diverse group into related subsamples to yield insights into their origin would be repeated again and again over the years, first by Minkowski when he sepa- rated supernovae of Type I (no hydrogen in their spectra) from Type II (have hydrogen)2, and then by Elias et al. -
Timescales for Detecting Magnetized White Dwarfs in Gravitational Wave Astronomy
Article Timescales for detecting magnetized white dwarfs in gravitational wave astronomy Surajit Kalita 1* 1 Department of Physics, Indian Institute of Science, Bangalore 560012, India; [email protected] * Correspondence: [email protected] Version February 20, 2021 submitted to Universe 1 Abstract: Over the past couple of decades, researchers have predicted more than a dozen 2 super-Chandrasekhar white dwarfs from the detections of over-luminous type Ia supernovae. 3 It turns out that magnetic fields and rotation can explain such massive white dwarfs. If these 4 rotating magnetized white dwarfs follow specific conditions, they can efficiently emit continuous 5 gravitational waves and various futuristic detectors, viz. LISA, BBO, DECIGO, and ALIA can detect 6 such gravitational waves with a significant signal-to-noise ratio. Moreover, we discuss various 7 timescales over which these white dwarfs can emit dipole and quadrupole radiations and show that 8 in the future, the gravitational wave detectors can directly detect the super-Chandrasekhar white 9 dwarfs depending on the magnetic field geometry and its strength. 10 Keywords: White dwarfs; gravitational waves; magnetic fields; rotation; luminosity 11 1. Introduction 12 Over the years, the luminosities of type Ia supernovae (SNeIa) are used as one of the standard 13 candles in cosmology to measure distances of various astronomical objects. This is due to the reason that 14 the peak luminosities of all the SNeIa are similar, as they are originated from the white dwarfs (WDs) 15 burst around the Chandrasekhar mass-limit (∼ 1.4M for carbon-oxygen non-rotating non-magnetized 16 WDs [1]). However, the detection of various over-luminous SNeIa, such as SN 2003fg [2], SN 2007if 17 [3], etc., for about a couple of decades, questions the complete validity of the standard candle using 18 SNeIa. -
The Ejected Mass Distribution of Type Ia Supernovae: a Significant Rate of Non-Chandrasekhar-Mass Progenitors
MNRAS 445, 2535–2544 (2014) doi:10.1093/mnras/stu1808 The ejected mass distribution of Type Ia supernovae: a significant rate of non-Chandrasekhar-mass progenitors R. A. Scalzo,1,2‹ A. J. Ruiter1,2,3 andS.A.Sim2,4 1Research School of Astronomy and Astrophysics, Australian National University, Canberra, ACT 2611, Australia 2ARC Centre of Excellence for All-Sky Astrophysics (CAASTRO), The Australian National University, Cotter Road, Weston Creek ACT 2611 Australia 3Max-Planck-Institut fur¨ Astrophysik, Karl-Schwarzschild-Str. 1, D-85741 Garching bei Munchen,¨ Germany 4Astrophysics Research Centre, School of Mathematics and Physics, Queen’s University Belfast, Belfast, BT7 1NN, UK Accepted 2014 September 1. Received 2014 August 21; in original form 2014 June 30 Downloaded from ABSTRACT The ejected mass distribution of Type Ia supernovae (SNe Ia) directly probes progenitor evolutionary history and explosion mechanisms, with implications for their use as cosmological probes. Although the Chandrasekhar mass is a natural mass scale for the explosion of white http://mnras.oxfordjournals.org/ dwarfs as SNe Ia, models allowing SNe Ia to explode at other masses have attracted much recent attention. Using an empirical relation between the ejected mass and the light-curve 56 width, we derive ejected masses Mej and Ni masses MNi for a sample of 337 SNe Ia with redshifts z<0.7 used in recent cosmological analyses. We use hierarchical Bayesian inference to reconstruct the joint Mej–MNi distribution, accounting for measurement errors. The inferred marginal distribution of Mej has a long tail towards sub-Chandrasekhar masses, but cuts off sharply above 1.4 M. -
The Type Ia Supernova SNLS-03D3bb from a Super-Chandrasekhar-Mass
The type Ia supernova SNLS-03D3bb from a super- Chandrasekhar-mass white dwarf star D. Andrew Howell1, Mark Sullivan1, Peter E. Nugent2, Richard S. Ellis3, Alexander J. Conley1, Damien Le Borgne4, Raymond G. Carlberg1, Julien Guy5, David Balam6, Stephane Basa7, Dominique Fouchez8, Isobel M. Hook9, Eric Y. Hsiao6, James D. Neill6, Reynald Pain5, Kathryn M. Perrett1, Christopher J. Pritchet6 1Department of Astronomy and Astrophysics, University of Toronto, 60 St. George Street, Toronto, ON M5S 3H8, Canada 2Lawrence Berkeley National Laboratory, Mail Stop 50-232, 1 Cyclotron Road, Berkeley CA 94720 USA 3California Institute of Technology, E. California Blvd., Pasadena, CA 91125, USA 4DAPNIA/Service d’Astrophysique, CEA/Saclay, 91191 Gif-sur-Yvette Cedex, France 5LPNHE, CNRS-IN2P3 and University of Paris VI & VII, 75005 Paris, France 6Department of Physics and Astronomy, University of Victoria, PO Box 3055, Victoria, BC V8W 3P6, Canada 7LAM CNRS, BP8, Traverse du Siphon, 13376 Marseille Cedex 12, France 8CPPM, CNRS-IN2P3 and University Aix Marseille II, Case 907, 13288 Marseille Cedex 9, France 9University of Oxford Astrophysics, Denys Wilkinson Building, Keble Road, Oxford OX1 3RH, UK The acceleration of the expansion of the universe, and the need for Dark Energy, were in- ferred from the observations of Type Ia supernovae (SNe Ia)1;2. There is consensus that SNe Ia are thermonuclear explosions that destroy carbon-oxygen white dwarf stars that accrete matter from a companion star3, although the nature of this companion remains uncertain. SNe Ia are thought to be reliable distance indicators because they have a standard amount of fuel and a uniform trigger — they are predicted to explode when the mass of the white dwarf nears the Chandrasekhar mass4 — 1.4 solar masses. -
Type Ia Supernovae As Stellar Endpoints and Cosmological Tools
REVIEW Published 14 Jun 2011 | DOI: 10.1038/ncomms1344 Type Ia supernovae as stellar endpoints and cosmological tools D. Andrew Howell1,2 Empirically, Type Ia supernovae are the most useful, precise, and mature tools for determining astronomical distances. Acting as calibrated candles they revealed the presence of dark energy and are being used to measure its properties. However, the nature of the Type Ia explosion, and the progenitors involved, have remained elusive, even after seven decades of research. But now, new large surveys are bringing about a paradigm shift—we can finally compare samples of hundreds of supernovae to isolate critical variables. As a result of this, and advances in modelling, breakthroughs in understanding all aspects of these supernovae are finally starting to happen. uest stars (who could have imagined they were distant stellar explosions?) have been surprising humans for at least 950 years, but probably far longer. They amazed and confounded the likes of Tycho, Kepler and Galileo, to name a few. But it was not until the separation of these events G 1 into novae and supernovae by Baade and Zwicky that progress understanding them began in earnest . This process of splitting a diverse group into related subsamples to yield insights into their origin would be repeated again and again over the years, first by Minkowski when he separated supernovae of Type I (no hydrogen in their spectra) from Type II (have hydrogen)2, and then by Elias et al. when they deter- mined that Type Ia supernovae (SNe Ia) were distinct3. We now define Type Ia supernovae as those without hydrogen or helium in their spectra, but with strong ionized silicon (SiII), which has observed absorption lines at 6,150, 5,800 and 4,000 Å (ref. -
Strong Near-Infrared Carbon in the Type Ia Supernova Iptf13ebh⋆⋆⋆
A&A 578, A9 (2015) Astronomy DOI: 10.1051/0004-6361/201425297 & c ESO 2015 Astrophysics Strong near-infrared carbon in the Type Ia supernova iPTF13ebh?;?? E. Y. Hsiao1;2, C. R. Burns3, C. Contreras2;1, P. Höflich4, D. Sand5, G. H. Marion6;7, M. M. Phillips2, M. Stritzinger1, S. González-Gaitán8;9, R. E. Mason10, G. Folatelli11;12, E. Parent13, C. Gall1;14, R. Amanullah15, G. C. Anupama16, I. Arcavi17;18, D. P. K. Banerjee19, Y. Beletsky2, G. A. Blanc3;9, J. S. Bloom20, P. J. Brown21, A. Campillay2, Y. Cao22, A. De Cia26, T. Diamond4, W. L. Freedman3, C. Gonzalez2, A. Goobar15, S. Holmbo1, D. A. Howell17;18, J. Johansson15, M. M. Kasliwal3, R. P. Kirshner7, K. Krisciunas21, S. R. Kulkarni22, K. Maguire24, P. A. Milne25, N. Morrell2, P. E. Nugent23;20, E. O. Ofek26, D. Osip2, P. Palunas2, D. A. Perley22, S. E. Persson3, A. L. Piro3, M. Rabus27, M. Roth2, J. M. Schiefelbein21, S. Srivastav16, M. Sullivan28, N. B. Suntzeff21, J. Surace29, P. R. Wo´zniak30, and O. Yaron26 (Affiliations can be found after the references) Received 7 November 2014 / Accepted 7 March 2015 ABSTRACT We present near-infrared (NIR) time-series spectroscopy, as well as complementary ultraviolet (UV), optical, and NIR data, of the Type Ia su- pernova (SN Ia) iPTF13ebh, which was discovered within two days from the estimated time of explosion. The first NIR spectrum was taken merely 2:3 days after explosion and may be the earliest NIR spectrum yet obtained of a SN Ia. The most striking features in the spectrum are several NIR C i lines, and the C i λ1.0693 µm line is the strongest ever observed in a SN Ia. -
General Relativity and Relativistic Astrophysics Arxiv:1609.01862V1
General relativity and relativistic astrophysics Banibrata Mukhopadhyay Department of Physics, Indian Institute of Science, Bangalore 560012, India [email protected] September 8, 2016 Article published for a special section in Current Science dedicated to 100 years of general relativity Abstract Einstein established the theory of general relativity and the corresponding field equation in 1915 and its vacuum solutions were obtained by Schwarzschild and Kerr for, respectively, static and rotating black holes, in 1916 and 1963, respec- tively. They are, however, still playing an indispensable role, even after 100 years of their original discovery, to explain high energy astrophysical phenomena. Ap- plication of the solutions of Einstein's equation to resolve astrophysical phenomena has formed an important branch, namely relativistic astrophysics. I devote this article to enlightening some of the current astrophysical problems based on general relativity. However, there seem to be some issues with regard to explaining certain astrophysical phenomena based on Einstein's theory alone. I show that Einstein's theory and its modified form, both are necessary to explain modern astrophysical processes, in particular, those related to compact objects. arXiv:1609.01862v1 [astro-ph.HE] 7 Sep 2016 1 1 Introduction Within a few months of the celebrated discovery of Einstein's field equation [1], Schwarzschild obtained its vacuum solution in spherical symmetry [2]. However, it took almost another half a century before Kerr obtained its vacuum solution for an axisymmetric spacetime [3], which was a very complicated job at that time. The former solution is very useful to understand the spacetime properties around a static black hole, called Schwarzschild black hole. -
The Birth Rate of Subluminous and Overluminous Type Ia Supernovae
A&A 525, A129 (2011) Astronomy DOI: 10.1051/0004-6361/201014967 & c ESO 2010 Astrophysics The birth rate of subluminous and overluminous type Ia supernovae X. C. Meng1,W.C.Chen2,3,W.M.Yang1,6, and Z. M. Li4,5 1 School of Physics and Chemistry, Henan Polytechnic University, Jiaozuo 454000, PR China e-mail: [email protected] 2 Department of Physics, Shangqiu Normal University, 476000 Shangqiu, PR China 3 Key Laboratory of Modern Astronomy and Astrophysics (Nanjing University), Ministry of Education, 210093 Nanjing, PR China 4 College of Physics and Electronic Information, Dali University, 671003 Dali, PR China 5 National Astronomical Observatories, Chinese Academy of Sciences, 100012 Beijing, PR China 6 Department of Astronomy, Beijing Normal University, 100875 Beijing, PR China Received 10 May 2010 / Accepted 24 October 2010 ABSTRACT Context. Based on the single degenerate (SD) scenario, a super-Chandrasekhar mass model derived from the rapid rotation of a progenitor star may account for the overluminous type Ia supernovae (SNe Ia) like SN 2003fg. Previous authors calculated a series of binary evolution and showed the parameter spaces for the super-Chandrasekhar mass model. Another team developed an equal-mass double degenerate (DD) model to explain subluminous SNe Ia like SN 1991bg. But they did not show the evolution of the birth rate of these peculiar SNe Ia or compared them with absolute birth rates from observations. Aims. We aim to show the evolution of the birth rates of these peculiar SNe Ia based on the results of these other works, and compare the birth rates with observations to check whether these model may account for all the peculiar SNe Ia. -
Defining Photometric Peculiar Type Ia Supernovae
Draft version September 18, 2018 A Preprint typeset using LTEX style emulateapj v. 12/16/11 DEFINING PHOTOMETRIC PECULIAR TYPE IA SUPERNOVAE S. Gonzalez-Gait´ an´ 1,2, E. Y. Hsiao3, G. Pignata4,1, F. Forster¨ 5,1, C. P. Gutierrez´ 1,2, F. Bufano1,4, L. Galbany1,2, G. Folatelli6, M. M. Phillips3, M. Hamuy2,1, J. P. Anderson7, and T. de Jaeger1,2 1Millennium Institute of Astrophysics, Casilla 36-D, Santiago, Chile 2Departamento de Astronom´ıa, Universidad de Chile, Camino El Observatorio 1515, Las Condes, Santiago, Chile 3Carnegie Observatories, Las Campanas Observatory, Casilla 601, La Serena, Chile 4Departamento de Ciencias F´ısicas, Universidad Andres Bello, Avda. Republica 252, Santiago, Chile 5Centro de Modelamiento Matem´atico, Universidad de Chile, Av. Blanco Encalada 2120 Piso 7, Santiago, Chile 6Kavli Institute for the Physics and Mathematics of the Universe, the University of Tokyo, Kashiwa, Japan 277-8583 (Kavli IPMU, WPI) and 7European Southern Observatory, Alonso de C´ordova 3107, Casilla 19, Santiago, Chile Draft version September 18, 2018 ABSTRACT We present a new photometric identification technique for SN 1991bg-like type Ia supernovae (SNe Ia), i.e. objects with light-curve characteristics such as later primary maxima and absence of secondary peak in redder filters. This method is capable of selecting out this sub-group from the normal type Ia population. Furthermore, we find that recently identified peculiar sub-types such as SNe Iax and super-Chandrasekhar SNe Ia have similar photometric characteristics as 91bg-like SNe Ia, namely the absence of secondary maxima and shoulders at longer wavelengths, and can also be classified with our technique.