Composition and Diversity of Medicago Truncatula Root Bacterial Endophyte Populations Resulting from Growth in Different Oklahoma Soils
Total Page:16
File Type:pdf, Size:1020Kb
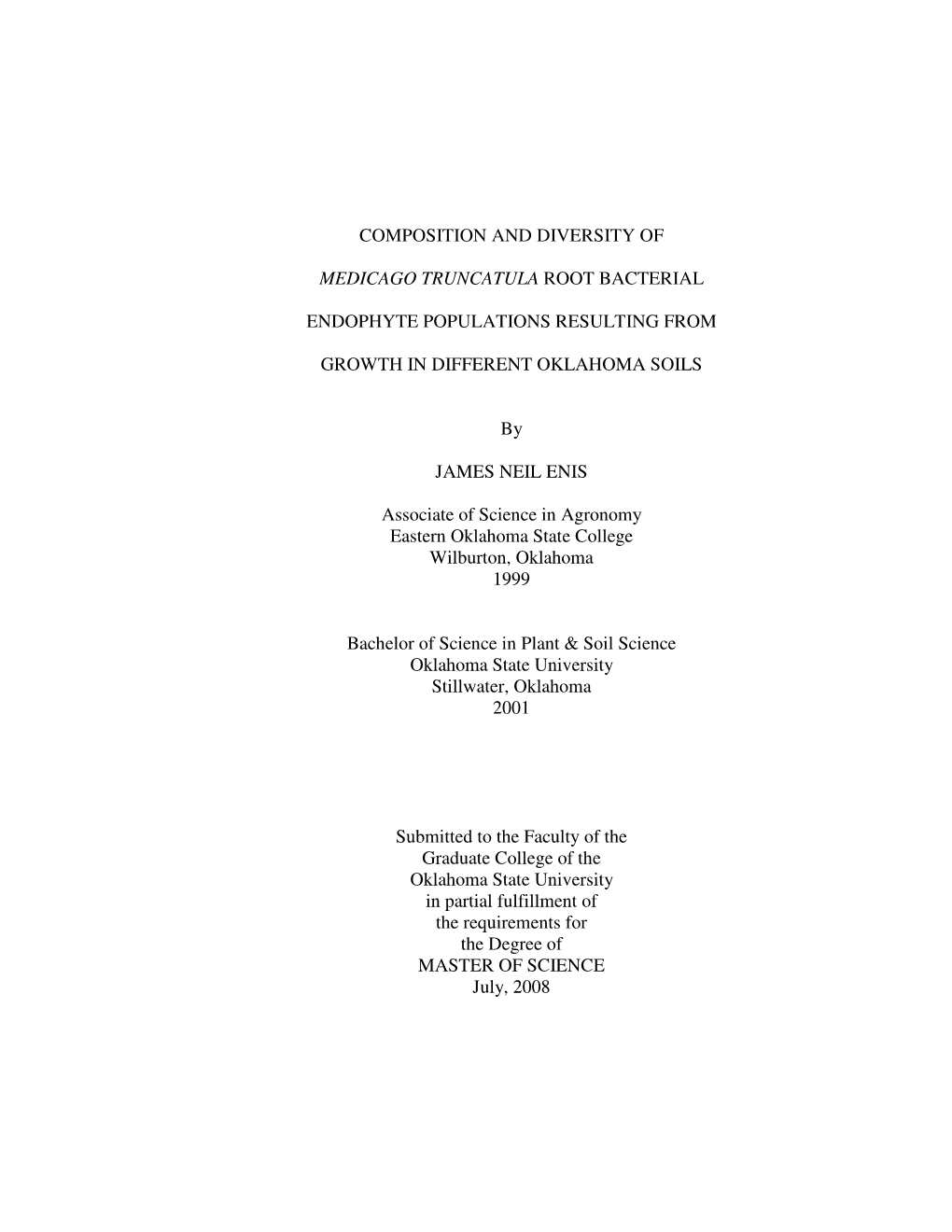
Load more
Recommended publications
-
The 2014 Golden Gate National Parks Bioblitz - Data Management and the Event Species List Achieving a Quality Dataset from a Large Scale Event
National Park Service U.S. Department of the Interior Natural Resource Stewardship and Science The 2014 Golden Gate National Parks BioBlitz - Data Management and the Event Species List Achieving a Quality Dataset from a Large Scale Event Natural Resource Report NPS/GOGA/NRR—2016/1147 ON THIS PAGE Photograph of BioBlitz participants conducting data entry into iNaturalist. Photograph courtesy of the National Park Service. ON THE COVER Photograph of BioBlitz participants collecting aquatic species data in the Presidio of San Francisco. Photograph courtesy of National Park Service. The 2014 Golden Gate National Parks BioBlitz - Data Management and the Event Species List Achieving a Quality Dataset from a Large Scale Event Natural Resource Report NPS/GOGA/NRR—2016/1147 Elizabeth Edson1, Michelle O’Herron1, Alison Forrestel2, Daniel George3 1Golden Gate Parks Conservancy Building 201 Fort Mason San Francisco, CA 94129 2National Park Service. Golden Gate National Recreation Area Fort Cronkhite, Bldg. 1061 Sausalito, CA 94965 3National Park Service. San Francisco Bay Area Network Inventory & Monitoring Program Manager Fort Cronkhite, Bldg. 1063 Sausalito, CA 94965 March 2016 U.S. Department of the Interior National Park Service Natural Resource Stewardship and Science Fort Collins, Colorado The National Park Service, Natural Resource Stewardship and Science office in Fort Collins, Colorado, publishes a range of reports that address natural resource topics. These reports are of interest and applicability to a broad audience in the National Park Service and others in natural resource management, including scientists, conservation and environmental constituencies, and the public. The Natural Resource Report Series is used to disseminate comprehensive information and analysis about natural resources and related topics concerning lands managed by the National Park Service. -
A Study on the Phototrophic Microbial Mat Communities of Sulphur Mountain Thermal Springs and Their Association with the Endangered, Endemic Snail Physella Johnsoni
A Study on the Phototrophic Microbial Mat Communities of Sulphur Mountain Thermal Springs and their Association with the Endangered, Endemic Snail Physella johnsoni By Michael Bilyj A thesis submitted to the Faculty of Graduate Studies in partial fulfillment of the requirements for the degree of Master of Science Department of Microbiology Faculty of Science University of Manitoba Winnipeg, Manitoba October 2011 © Copyright 2011, Michael A. Bilyj 1 Abstract The seasonal population fluctuation of anoxygenic phototrophs and the diversity of cyanobacteria at the Sulphur Mountain thermal springs of Banff, Canada were investigated and compared to the drastic population changes of the endangered snail Physella johnsoni. A new species and two strains of Rhodomicrobium were taxonomically characterized in addition to new species of Rhodobacter and Erythromicrobium. Major mat-forming organisms included Thiothrix-like species, oxygenic phototrophs of genera Spirulina, Oscillatoria, and Phormidium and purple nonsulfur bacteria Rhodobacter, Rhodopseudomonas and Rhodomicrobium. Aerobic anoxygenic phototrophs comprised upwards of 9.6 x 104 CFU/cm2 of mat or 18.9% of total aerobic heterotrophic bacterial isolates at certain sites, while maximal purple nonsulfur and purple sulfur bacteria were quantified at 3.2 x 105 and 2.0 x 106 CFU/cm2 of mat, respectively. Photosynthetic activity measurements revealed incredibly productive carbon fixation rates averaging 40.5 mg C/cm2/24 h. A temporal mismatch was observed for mat area and prokaryote-based organics to P. johnsoni population flux in a ―tracking inertia‖ manner. 2 Acknowledgements It is difficult to express sufficient gratitude to my supervisor Dr. Vladimir Yurkov for his unfaltering patience, generosity and motivation throughout this entire degree. -
Alpine Soil Bacterial Community and Environmental Filters Bahar Shahnavaz
Alpine soil bacterial community and environmental filters Bahar Shahnavaz To cite this version: Bahar Shahnavaz. Alpine soil bacterial community and environmental filters. Other [q-bio.OT]. Université Joseph-Fourier - Grenoble I, 2009. English. tel-00515414 HAL Id: tel-00515414 https://tel.archives-ouvertes.fr/tel-00515414 Submitted on 6 Sep 2010 HAL is a multi-disciplinary open access L’archive ouverte pluridisciplinaire HAL, est archive for the deposit and dissemination of sci- destinée au dépôt et à la diffusion de documents entific research documents, whether they are pub- scientifiques de niveau recherche, publiés ou non, lished or not. The documents may come from émanant des établissements d’enseignement et de teaching and research institutions in France or recherche français ou étrangers, des laboratoires abroad, or from public or private research centers. publics ou privés. THÈSE Pour l’obtention du titre de l'Université Joseph-Fourier - Grenoble 1 École Doctorale : Chimie et Sciences du Vivant Spécialité : Biodiversité, Écologie, Environnement Communautés bactériennes de sols alpins et filtres environnementaux Par Bahar SHAHNAVAZ Soutenue devant jury le 25 Septembre 2009 Composition du jury Dr. Thierry HEULIN Rapporteur Dr. Christian JEANTHON Rapporteur Dr. Sylvie NAZARET Examinateur Dr. Jean MARTIN Examinateur Dr. Yves JOUANNEAU Président du jury Dr. Roberto GEREMIA Directeur de thèse Thèse préparée au sien du Laboratoire d’Ecologie Alpine (LECA, UMR UJF- CNRS 5553) THÈSE Pour l’obtention du titre de Docteur de l’Université de Grenoble École Doctorale : Chimie et Sciences du Vivant Spécialité : Biodiversité, Écologie, Environnement Communautés bactériennes de sols alpins et filtres environnementaux Bahar SHAHNAVAZ Directeur : Roberto GEREMIA Soutenue devant jury le 25 Septembre 2009 Composition du jury Dr. -
Which Organisms Are Used for Anti-Biofouling Studies
Table S1. Semi-systematic review raw data answering: Which organisms are used for anti-biofouling studies? Antifoulant Method Organism(s) Model Bacteria Type of Biofilm Source (Y if mentioned) Detection Method composite membranes E. coli ATCC25922 Y LIVE/DEAD baclight [1] stain S. aureus ATCC255923 composite membranes E. coli ATCC25922 Y colony counting [2] S. aureus RSKK 1009 graphene oxide Saccharomycetes colony counting [3] methyl p-hydroxybenzoate L. monocytogenes [4] potassium sorbate P. putida Y. enterocolitica A. hydrophila composite membranes E. coli Y FESEM [5] (unspecified/unique sample type) S. aureus (unspecified/unique sample type) K. pneumonia ATCC13883 P. aeruginosa BAA-1744 composite membranes E. coli Y SEM [6] (unspecified/unique sample type) S. aureus (unspecified/unique sample type) graphene oxide E. coli ATCC25922 Y colony counting [7] S. aureus ATCC9144 P. aeruginosa ATCCPAO1 composite membranes E. coli Y measuring flux [8] (unspecified/unique sample type) graphene oxide E. coli Y colony counting [9] (unspecified/unique SEM sample type) LIVE/DEAD baclight S. aureus stain (unspecified/unique sample type) modified membrane P. aeruginosa P60 Y DAPI [10] Bacillus sp. G-84 LIVE/DEAD baclight stain bacteriophages E. coli (K12) Y measuring flux [11] ATCC11303-B4 quorum quenching P. aeruginosa KCTC LIVE/DEAD baclight [12] 2513 stain modified membrane E. coli colony counting [13] (unspecified/unique colony counting sample type) measuring flux S. aureus (unspecified/unique sample type) modified membrane E. coli BW26437 Y measuring flux [14] graphene oxide Klebsiella colony counting [15] (unspecified/unique sample type) P. aeruginosa (unspecified/unique sample type) graphene oxide P. aeruginosa measuring flux [16] (unspecified/unique sample type) composite membranes E. -
The Gut Microbiome of the Sea Urchin, Lytechinus Variegatus, from Its Natural Habitat Demonstrates Selective Attributes of Micro
FEMS Microbiology Ecology, 92, 2016, fiw146 doi: 10.1093/femsec/fiw146 Advance Access Publication Date: 1 July 2016 Research Article RESEARCH ARTICLE The gut microbiome of the sea urchin, Lytechinus variegatus, from its natural habitat demonstrates selective attributes of microbial taxa and predictive metabolic profiles Joseph A. Hakim1,†, Hyunmin Koo1,†, Ranjit Kumar2, Elliot J. Lefkowitz2,3, Casey D. Morrow4, Mickie L. Powell1, Stephen A. Watts1,∗ and Asim K. Bej1,∗ 1Department of Biology, University of Alabama at Birmingham, 1300 University Blvd, Birmingham, AL 35294, USA, 2Center for Clinical and Translational Sciences, University of Alabama at Birmingham, Birmingham, AL 35294, USA, 3Department of Microbiology, University of Alabama at Birmingham, Birmingham, AL 35294, USA and 4Department of Cell, Developmental and Integrative Biology, University of Alabama at Birmingham, 1918 University Blvd., Birmingham, AL 35294, USA ∗Corresponding authors: Department of Biology, University of Alabama at Birmingham, 1300 University Blvd, CH464, Birmingham, AL 35294-1170, USA. Tel: +1-(205)-934-8308; Fax: +1-(205)-975-6097; E-mail: [email protected]; [email protected] †These authors contributed equally to this work. One sentence summary: This study describes the distribution of microbiota, and their predicted functional attributes, in the gut ecosystem of sea urchin, Lytechinus variegatus, from its natural habitat of Gulf of Mexico. Editor: Julian Marchesi ABSTRACT In this paper, we describe the microbial composition and their predictive metabolic profile in the sea urchin Lytechinus variegatus gut ecosystem along with samples from its habitat by using NextGen amplicon sequencing and downstream bioinformatics analyses. The microbial communities of the gut tissue revealed a near-exclusive abundance of Campylobacteraceae, whereas the pharynx tissue consisted of Tenericutes, followed by Gamma-, Alpha- and Epsilonproteobacteria at approximately equal capacities. -
Biodegradation of Fluorinated Compounds Widely Used in Agro-Industrial Applications Diogo Alves Da Mota Alexandrino M 2016
DISSERTAÇÃO DE MESTRADO TOXICOLOGIA E CONTAMINAÇÃO AMBIENTAIS Biodegradation of fluorinated compounds widely used in agro-industrial applications Diogo Alves da Mota Alexandrino M 2016 Diogo Alves da Mota Alexandrino BIODEGRADATION OF FLUORINATED COMPOUNDS WIDELY USED IN AGRO-INDUSTRIAL CONTEXTS Dissertação de Candidatura ao grau de Mestre em Toxicologia e Contaminação Ambientais submetida ao Instituto de Ciências Biomédicas de Abel Salazar da Universidade do Porto. Orientadora – Doutora Maria de Fátima Carvalho Categoria – Investigadora Auxiliar Afiliação – Centro Interdisciplinar de Investigação Marinha e Ambiental da Universidade do Porto Co-orientadora – Doutora Ana Paula Mucha Categoria – Investigadora Auxiliar Afiliação – Centro Interdisciplinar de Investigação Marinha e Ambiental da Universidade do Porto ACKNOWLEDGEMENTS Firstly, I would like to thank my supervisor, Dr. Maria F. Carvalho, without whom the work integrated in this thesis would have not been possible. I genuinely thank her incredible dedication and trust, certain that part of my future goals have been established as a result of her mentoring, which became both an incredible honour and a fundamental phase in my personal and professional development. I would also like to thank my co-supervisor, Dr. Ana Paula Mucha, to whom I thank for the opportunity of integrating her laboratory, where I was always given all the conditions to develop my work to the fullest of its potential. Secondly, I would like to acknowledge CIIMAR - Interdisciplinary Centre of Marine and Environmental Research and Departamento de Química e Bioquímica of Faculty of Sciences of University of Porto, for the use of all the equipment, installations and facilities. To my lab mates at Ecobiotec (CIIMAR-UP), I recognise their friendship, as well as all the input in my work and precious help and support, with a special emphasis to Patricia Duarte, Filipa Santos, Joana Fernandes and Inês Ribeiro. -
17, 3203–3222, 2020 © Author(S) 2020
Biogeosciences, 17, 3203–3222, 2020 https://doi.org/10.5194/bg-17-3203-2020 © Author(s) 2020. This work is distributed under the Creative Commons Attribution 4.0 License. The contribution of microbial communities in polymetallic nodules to the diversity of the deep-sea microbiome of the Peru Basin (4130–4198 m depth) Massimiliano Molari1, Felix Janssen1,2, Tobias R. Vonnahme1,a, Frank Wenzhöfer1,2, and Antje Boetius1,2 1Max Planck Institute for Marine Microbiology, Bremen, Germany 2HGF MPG Joint Research Group for Deep-Sea Ecology and Technology, Alfred Wegener Institute for Polar and Marine Research, Bremerhaven, Germany apresent address: UiT the Arctic University of Tromsø, Tromsø, Norway Correspondence: Massimiliano Molari ([email protected]) Received: 16 January 2020 – Discussion started: 3 February 2020 Revised: 27 April 2020 – Accepted: 15 May 2020 – Published: 25 June 2020 Abstract. Industrial-scale mining of deep-sea polymetal- tween the Clarion–Clipperton Fracture Zone (CCZ) and the lic nodules will remove nodules in large areas of the sea Peru Basin suggest that changes in environmental setting floor. The regrowth of the nodules by metal precipita- (e.g. sedimentation rates) also play a significant role in struc- tion is estimated to take millions of years. Thus, for fu- turing the nodule microbiome. ture mining impact studies, it is crucial to understand the role of nodules in shaping microbial diversity and function in deep-sea environments. Here we investigated microbial- community composition based on 16S rRNA gene sequences 1 Introduction retrieved from sediments and nodules of the Peru Basin (4130–4198 m water depth). The nodule field of the Peru Polymetallic nodules (or manganese nodules) occur in Basin showed a typical deep-sea microbiome, with domi- abyssal plains (4000–6000 m water depth) and consist pri- nance of the classes Gammaproteobacteria, Alphaproteobac- marily of manganese and iron as well as many other metals teria, Deltaproteobacteria, and Acidimicrobiia. -
University of Groningen Labrys Portucalensis Sp Nov., A
University of Groningen Labrys portucalensis sp nov., a fluorobenzene-degrading bacterium isolated from an industrially contaminated sediment in northern Portugal Carvalho, Maria F.; De Marco, Paolo; Duque, Anouk F.; Pacheco, Catarina C.; Janssen, Dick; Castro, Paula M. L. Published in: International Journal of Systematic and Evolutionary Microbiology DOI: 10.1099/ijs.0.65472-0 IMPORTANT NOTE: You are advised to consult the publisher's version (publisher's PDF) if you wish to cite from it. Please check the document version below. Document Version Publisher's PDF, also known as Version of record Publication date: 2008 Link to publication in University of Groningen/UMCG research database Citation for published version (APA): Carvalho, M. F., De Marco, P., Duque, A. F., Pacheco, C. C., Janssen, D. B., & Castro, P. M. L. (2008). Labrys portucalensis sp nov., a fluorobenzene-degrading bacterium isolated from an industrially contaminated sediment in northern Portugal. International Journal of Systematic and Evolutionary Microbiology, 58(3), 692-698. DOI: 10.1099/ijs.0.65472-0 Copyright Other than for strictly personal use, it is not permitted to download or to forward/distribute the text or part of it without the consent of the author(s) and/or copyright holder(s), unless the work is under an open content license (like Creative Commons). Take-down policy If you believe that this document breaches copyright please contact us providing details, and we will remove access to the work immediately and investigate your claim. Downloaded from the University of Groningen/UMCG research database (Pure): http://www.rug.nl/research/portal. For technical reasons the number of authors shown on this cover page is limited to 10 maximum. -
Deoxyribonucleic Acid Base Sequence Homologies of Some Budding and Prosthecate Bacterla RICHARD L
JOURNAL OF BACTERIOLOGY, Apr. 1972, p. 256-261 Vol. 110, No. 1 Copyright © 1972 American Society for Microbiology Printed in U.S.A. Deoxyribonucleic Acid Base Sequence Homologies of Some Budding and Prosthecate Bacterla RICHARD L. MOORE' AND PETER HIRSCH2 Department of Microbiology and Public Health, Michigan State University, East Lansing, Michigan 48823 Received for publication 21 December 1971 The genetic relatedness of a number of budding and prosthecate bacteria was determined by deoxyribonucleic acid (DNA) homology experiments of the di- rect binding type. Strains of Hyphomicrobium sp. isolated from aquatic habi- tats were found to have relatedness values ranging from 9 to 70% with strain "EA-617," a subculture of the Hyphomicrobium isolated by Mevius from river water. Strains obtained from soil enrichments had lower values with EA-617, ranging from 3 to 5%. Very little or no homology was detected between the amino acid-utilizing strain Hyphomicrobium neptunium and other Hyphomi- crobium strains, although significant homology was observed with the two Hyphomonas strains examined. No homology could be detected between pros- thecate bacteria of the genera Rhodomicrobium, Prosthecomicrobium, Ancal- omicrobium, or Caulobacter, and Hyphomicrobium strain EA-617 or H. nep- tunium LE-670. The grouping of Hyphomicrobium strains by their relatedness values agrees well with a grouping according to the base composition of their DNA species. It is concluded that bacteria possessing cellular extensions repre- sent a widely diverse group of organisms. Two genera of bacteria, Hyphomicrobium drum, P. pneumaticum, Ancalomicrobium adetum, and Rhodomicrobium, are listed under the and Caulobacter crescentus were obtained from J. T. family of Hyphomicrobiaceae in the seventh Staley (Seattle); Rhodomicrobium vannielii was re- edition of Bergey's Manual of Determinative ceived from H. -
Appendices Physico-Chemical
http://researchcommons.waikato.ac.nz/ Research Commons at the University of Waikato Copyright Statement: The digital copy of this thesis is protected by the Copyright Act 1994 (New Zealand). The thesis may be consulted by you, provided you comply with the provisions of the Act and the following conditions of use: Any use you make of these documents or images must be for research or private study purposes only, and you may not make them available to any other person. Authors control the copyright of their thesis. You will recognise the author’s right to be identified as the author of the thesis, and due acknowledgement will be made to the author where appropriate. You will obtain the author’s permission before publishing any material from the thesis. An Investigation of Microbial Communities Across Two Extreme Geothermal Gradients on Mt. Erebus, Victoria Land, Antarctica A thesis submitted in partial fulfilment of the requirements for the degree of Master’s Degree of Science at The University of Waikato by Emily Smith Year of submission 2021 Abstract The geothermal fumaroles present on Mt. Erebus, Antarctica, are home to numerous unique and possibly endemic bacteria. The isolated nature of Mt. Erebus provides an opportunity to closely examine how geothermal physico-chemistry drives microbial community composition and structure. This study aimed at determining the effect of physico-chemical drivers on microbial community composition and structure along extreme thermal and geochemical gradients at two sites on Mt. Erebus: Tramway Ridge and Western Crater. Microbial community structure and physico-chemical soil characteristics were assessed via metabarcoding (16S rRNA) and geochemistry (temperature, pH, total carbon (TC), total nitrogen (TN) and ICP-MS elemental analysis along a thermal gradient 10 °C–64 °C), which also defined a geochemical gradient. -
Advance View Proofs
Microbes Environ. Vol. 00, No. 0, 000-000, 2015 https://www.jstage.jst.go.jp/browse/jsme2 doi:10.1264/jsme2.ME14123 Bacterial Community Analysis of Drinking Water Biofilms in Southern Sweden KATHARINA LÜHRIG1,2, BJÖRN CANBÄCK3, CATHERINE J. PAUL1,4, TOMAS JOHANSSON3, KENNETH M. PERSSON2,4, and PETER RÅDSTRÖM1* 1Applied Microbiology, Department of Chemistry, Lund University, P.O. Box 124, SE-221 00 Lund, Sweden; 2Sydvatten AB, Hyllie Stationstorg 21, SE-215 32 Malmö, Sweden; 3Microbial Ecology Group, Department of Biology, Lund University, Sölvegatan 37, SE-223 62 Lund, Sweden; and 4Water Resources Engineering, Department of Building and Environmental Technology, Lund University, P.O. Box 118, SE-221 00 Lund, Sweden (Received August 28, 2014—Accepted January 10, 2015—Published online February 21, 2015) Next-generation sequencing of the V1-V2 and V3 variable regions of the 16S rRNA gene generated a total of 674,116 reads that described six distinct bacterial biofilm communities from both water meters and pipes. A high degree of reproducibility was demonstrated for the experimental and analytical work-flow by analyzing the communities present in parallel water meters, the rare occurrence of biological replicates within a working drinking water distribution system. The communities observed in water meters from households that did not complain about their drinking water were defined by sequences representing Proteobacteria (82–87%), with 22–40% of all sequences being classified as Sphingomonadaceae. However, a water meter biofilm community from a household with consumer reports of red water and flowing water containing elevated levels of iron and manganese had fewer sequences representing Proteobacteria (44%); only 0.6% of all sequences were classified as Sphingomonadaceae; and, in contrast to the other water meter communities, markedly more sequences represented Nitrospira and Pedomicrobium. -
2010.-Hungria-MLI.Pdf
Mohammad Saghir Khan l Almas Zaidi Javed Musarrat Editors Microbes for Legume Improvement SpringerWienNewYork Editors Dr. Mohammad Saghir Khan Dr. Almas Zaidi Aligarh Muslim University Aligarh Muslim University Fac. Agricultural Sciences Fac. Agricultural Sciences Dept. Agricultural Microbiology Dept. Agricultural Microbiology 202002 Aligarh 202002 Aligarh India India [email protected] [email protected] Prof. Dr. Javed Musarrat Aligarh Muslim University Fac. Agricultural Sciences Dept. Agricultural Microbiology 202002 Aligarh India [email protected] This work is subject to copyright. All rights are reserved, whether the whole or part of the material is concerned, specifically those of translation, reprinting, re-use of illustrations, broadcasting, reproduction by photocopying machines or similar means, and storage in data banks. Product Liability: The publisher can give no guarantee for all the information contained in this book. The use of registered names, trademarks, etc. in this publication does not imply, even in the absence of a specific statement, that such names are exempt from the relevant protective laws and regulations and therefore free for general use. # 2010 Springer-Verlag/Wien Printed in Germany SpringerWienNewYork is a part of Springer Science+Business Media springer.at Typesetting: SPI, Pondicherry, India Printed on acid-free and chlorine-free bleached paper SPIN: 12711161 With 23 (partly coloured) Figures Library of Congress Control Number: 2010931546 ISBN 978-3-211-99752-9 e-ISBN 978-3-211-99753-6 DOI 10.1007/978-3-211-99753-6 SpringerWienNewYork Preface The farmer folks around the world are facing acute problems in providing plants with required nutrients due to inadequate supply of raw materials, poor storage quality, indiscriminate uses and unaffordable hike in the costs of synthetic chemical fertilizers.