Diversion of Flow and Sediment Toward a Side Channel Separated from the River by a LTD
Total Page:16
File Type:pdf, Size:1020Kb
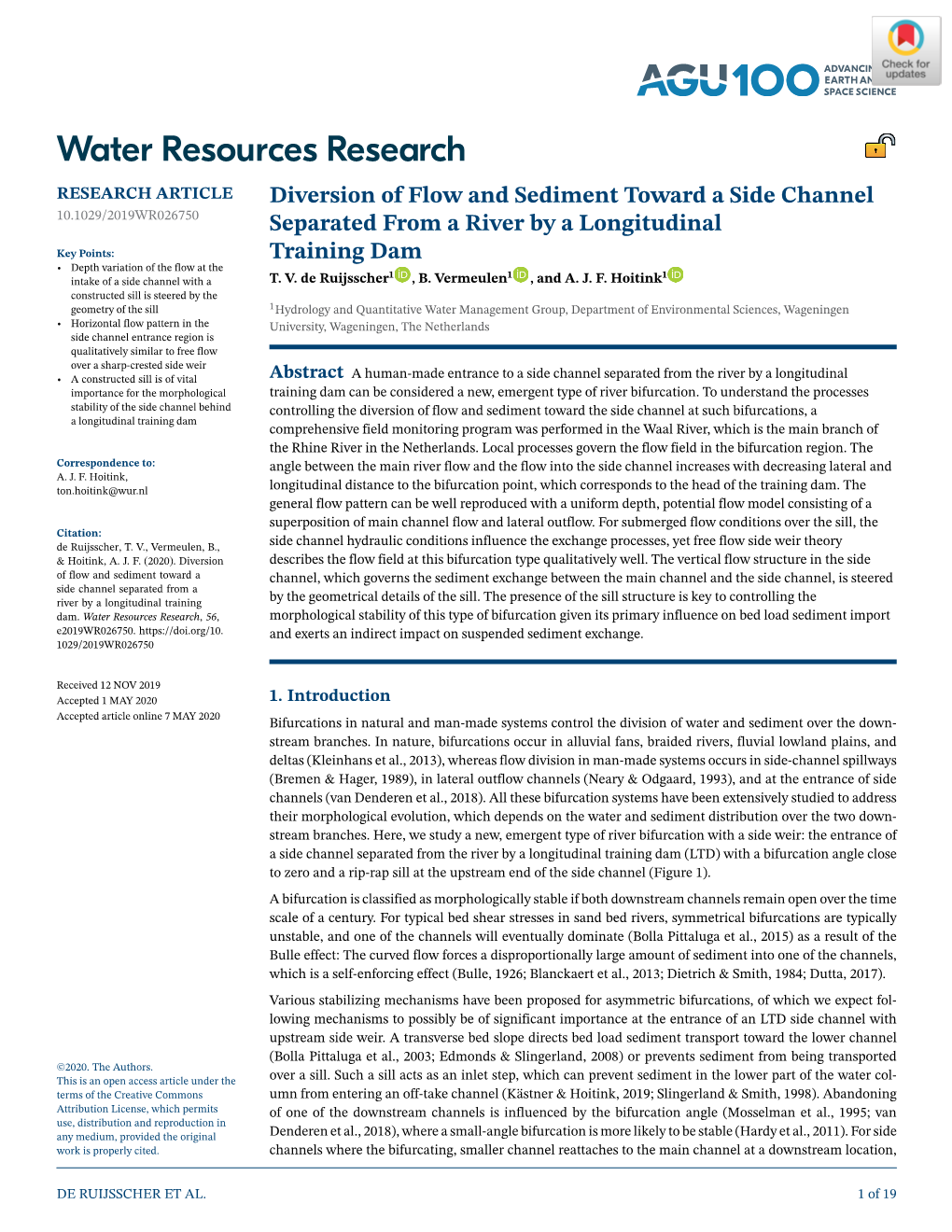
Load more
Recommended publications
-
Geomorphological Change and River Rehabilitation Alterra Is the Main Dutch Centre of Expertise on Rural Areas and Water Management
Geomorphological Change and River Rehabilitation Alterra is the main Dutch centre of expertise on rural areas and water management. It was founded 1 January 2000. Alterra combines a huge range of expertise on rural areas and their sustainable use, including aspects such as water, wildlife, forests, the environment, soils, landscape, climate and recreation, as well as various other aspects relevant to the development and management of the environment we live in. Alterra engages in strategic and applied research to support design processes, policymaking and management at the local, national and international level. This includes not only innovative, interdisciplinary research on complex problems relating to rural areas, but also the production of readily applicable knowledge and expertise enabling rapid and adequate solutions to practical problems. The many themes of Alterra’s research effort include relations between cities and their surrounding countryside, multiple use of rural areas, economy and ecology, integrated water management, sustainable agricultural systems, planning for the future, expert systems and modelling, biodiversity, landscape planning and landscape perception, integrated forest management, geoinformation and remote sensing, spatial planning of leisure activities, habitat creation in marine and estuarine waters, green belt development and ecological webs, and pollution risk assessment. Alterra is part of Wageningen University and Research centre (Wageningen UR) and includes two research sites, one in Wageningen and one on the island of Texel. Geomorphological Change and River Rehabilitation Case Studies on Lowland Fluvial Systems in the Netherlands H.P. Wolfert ALTERRA SCIENTIFIC CONTRIBUTIONS 6 ALTERRA GREEN WORLD RESEARCH, WAGENINGEN 2001 This volume was also published as a PhD Thesis of Utrecht University Promotor: Prof. -
Evolution of a New Tidal River Bifurcation: Dept
1: Universiteit Utrecht Faculty of Geosciences Evolution of a new tidal river bifurcation: Dept. Physical Geography [email protected] numerical modelling of an avulsion after a catastrophic storm surge 2: TNO Built Environment 1 2 1 and Geosciences / Geological Maarten Kleinhans , Henk Weerts , Kim Cohen Survey of The Netherlands Innundation was caused by a combination of storm surges, river floods and poor dike maintenance. Here we address: • How does an avulsion splay develop? • Is there simultaneous erosion upstream in the feeding channel? Biesbosch area, The Netherlands Reconstructed splay development Modelled splay development: (Zonneveld 1960) No tides, shallow basin, EH Tides, shallow basin, EH cum. erosion/sedimentation (m) cum. erosion/sedimentation (m) 07-Apr-1456 04:40:00 07-Apr-1456 04:40:00 12000 12000 old bifurcate silts up old bifurcate silts up Rhine 11000 11000 to avulsion locations Niie 10000 10000 eu → → w (R e M ) ) ot a m m tte a ( ( erd s 1421-1424: 9000 9000 a e e m) t t ) a a polder n n • 2 storm surges i i d d r 8000 r 8000 reclaimed o o • 2 river floods o o c c • poor dike maintenance by 1461 y 7000 y 7000 splay ¡ splay inundation from 2 sides 6000 1456 6000 1456 5000 5000 1.2 1.4 1.6 1.8 2 2.2 2.4 1.2 1.4 1.6 1.8 2 2.2 2.4 → 4 → 4 x coordinate (m) x 10 x coordinate (m) x 10 Haringvliet -4 -3 -2 -1 0 1 2 3 4 -4 -3 -2 -1 0 1 2 3 4 Haringvliet cum. -
Dynamic Flood Topographies in the Terai Region of Nepal
Edinburgh Research Explorer Dynamic flood topographies in the Terai region of Nepal Citation for published version: Dingle, EH, Creed, MJ, Sinclair, HD, Gautam, D, Gourmelen, N, Borthwick, AGL & Attal, M 2020, 'Dynamic flood topographies in the Terai region of Nepal', Earth Surface Processes and Landforms, vol. 45, no. 13, pp. 3092-3102. https://doi.org/10.1002/esp.4953 Digital Object Identifier (DOI): 10.1002/esp.4953 Link: Link to publication record in Edinburgh Research Explorer Document Version: Peer reviewed version Published In: Earth Surface Processes and Landforms General rights Copyright for the publications made accessible via the Edinburgh Research Explorer is retained by the author(s) and / or other copyright owners and it is a condition of accessing these publications that users recognise and abide by the legal requirements associated with these rights. Take down policy The University of Edinburgh has made every reasonable effort to ensure that Edinburgh Research Explorer content complies with UK legislation. If you believe that the public display of this file breaches copyright please contact [email protected] providing details, and we will remove access to the work immediately and investigate your claim. Download date: 01. Oct. 2021 Dynamic flood topographies in the Terai region of Nepal E.H. Dingle1,2#, M.J. Creed2, H.D. Sinclair2, D. Gautam3, N. Gourmelen2, A.G.L. Borthwick4, M. Attal2 1Department of Geography, Simon Fraser University, Burnaby, BC, Canada 2School of GeoSciences, University of Edinburgh, Drummond Street, -
Seismic Interpretation of Cree Sand Channels on the Scotian Shelf
Seismic interpretation of Cree Sand channels on the Scotian Shelf Rustam Khoudaiberdiev*, Craig Bennett, Paritosh Bhatnagar, and Sumit Verma The University of Texas of the Permian Basin Summary The Cree Sand of the Logan Canyon Formation is located at an approximated depth of 5,100 to 6,600 ft, with an overall Potential reservoirs can be found within deltaic channels, thickness of 1,500 ft (Figure 2). these channels have the ability to form continuous transport systems for hydrocarbons. Distributary sand-filled channels in particular can serve as excellent reservoirs. The emphasis of this study is taking a detailed look into the sand channels within the Cree Sand of the Logan Canyon, as well as using coherence and coherent energy seismic attributes to delineate these features. Extensive studies have been performed in analysis of deltaic channel systems and their ability to act as reservoirs for hydrocarbons. The paper will follow an equivalent approach, employing 3D seismic survey data and seismic interpretation techniques to identify and map sand channels. The study area is focused on the Penobscot field, located off of the eastern shores of Nova Scotia. Figure 1(a). Location map of the study area (Google Earth Maps). Introduction The Penobscot 3D seismic survey is displayed in the yellow rectangle. Deltaic channel systems are among the largest reservoirs for petroleum exploration in submarine environments. Distributary channels are formed through river bifurcation, Building of the Scotian Basin began soon after the separation or the splitting of a single river flow into multiple streams. and rifting of the North American continent from the African As sediment flows into the delta, the coarsest sediment is continent, all during the break-up of Pangea. -
Chute Channels in Large, Sand-Bed Meandering Rivers
Dynamics and Morphodynamic Implications of Chute Channels in Large, Sand-Bed Meandering Rivers Michael C. Grenfell Doctor of Philosophy in Geography College of Life and Environmental Sciences July 2012 Dynamics and Morphodynamic Implications of Chute Channels in Large, Sand-Bed Meandering Rivers Submitted by Michael Cyril Grenfell to the University of Exeter as a thesis for the degree of Doctor of Philosophy in Geography in the College of Life and Environmental Sciences July 2012 This thesis is available for Library use on the understanding that it is copyright material and that no quotation from the thesis may be published without proper acknowledgement. I certify that all material in this thesis which is not my own work has been identified, and that no material has previously been submitted and approved for the award of a degree by this or any other University. Michael Cyril Grenfell 2 ABSTRACT Chute channel formation is a key process in the transition from a single-thread meandering to a braided channel pattern, but the physical mechanisms driving the process remain unclear. This research combines GIS and spatial statistical analyses, field survey, Delft3D hydrodynamic and morphodynamic modelling, and Pb-210 alpha-geochronology, to investigate controls on chute initiation and stability, and the role of chute channels in the planform dynamics of large, sand-bed meandering rivers. Sand-bed reaches of four large, tropical rivers form the focus of detailed investigations; the Strickland and Ok Tedi in Papua New Guinea, the Beni in Bolivia, and the lower Paraguay on the Paraguay/Argentina border. Binary logistic regression analysis identifies bend migration style as a key control on chute channel initiation, with most chute channels forming at bends that are subject to a rapid rate of extension (elongation in a direction perpendicular to the valley axis). -
Sediment Load and Distribution in the Lower Skagit River, Skagit County, Washington
A Study by the U.S. Geological Survey Coastal Habitats in Puget Sound (CHIPS) Project Sediment Load and Distribution in the Lower Skagit River, Skagit County, Washington Scientific Investigations Report 2016–5106 U.S. Department of the Interior U.S. Geological Survey Front Cover: Downstream view of the Skagit River near Mount Vernon, Washington, September 28, 2012. Photograph by Eric Grossman, U.S. Geological Survey. Back Cover: Downstream view of the North Fork Skagit River at the bifurcation near Skagit City, Washington, September 14, 2012. Photograph by Eric Grossman, U.S. Geological Survey. Sediment Load and Distribution in the Lower Skagit River, Skagit County, Washington By Christopher A. Curran, Eric E. Grossman, Mark C. Mastin, and Raegan L. Huffman A Study by the U.S. Geological Survey Coastal Habitats in Puget Sound (CHIPS) Project Scientific Investigations Report 2016–5106 U.S. Department of the Interior U.S. Geological Survey U.S. Department of the Interior SALLY JEWELL, Secretary U.S. Geological Survey Suzette M. Kimball, Director U.S. Geological Survey, Reston, Virginia: 2016 For more information on the USGS—the Federal source for science about the Earth, its natural and living resources, natural hazards, and the environment—visit http://www.usgs.gov or call 1–888–ASK–USGS. For an overview of USGS information products, including maps, imagery, and publications, visit http://store.usgs.gov. Any use of trade, firm, or product names is for descriptive purposes only and does not imply endorsement by the U.S. Government. Although this information product, for the most part, is in the public domain, it also may contain copyrighted materials as noted in the text. -
University of Southampton Research Repository Eprints Soton
University of Southampton Research Repository ePrints Soton Copyright © and Moral Rights for this thesis are retained by the author and/or other copyright owners. A copy can be downloaded for personal non-commercial research or study, without prior permission or charge. This thesis cannot be reproduced or quoted extensively from without first obtaining permission in writing from the copyright holder/s. The content must not be changed in any way or sold commercially in any format or medium without the formal permission of the copyright holders. When referring to this work, full bibliographic details including the author, title, awarding institution and date of the thesis must be given e.g. AUTHOR (year of submission) "Full thesis title", University of Southampton, name of the University School or Department, PhD Thesis, pagination http://eprints.soton.ac.uk UNIVERSITY OF SOUTHAMPTON FACULTY OF SOCIAL AND HUMAN SCIENCES Geography and Environment Channel Planform Dynamics of the Ganga-Padma System, India by Niladri Gupta Thesis for the degree of Doctor of Philosophy May 2012 UNIVERSITY OF SOUTHAMPTON ABSTRACT FACULTY OF SOCIAL AND HUMAN SCIENCES Geography and Environment Doctor of Philosophy CHANNEL PLANFORM DYNAMICS OF THE GANGA-PADMA SYSTEM, INDIA by Niladri Gupta The Landsat programme, which started in 1972, initiated an era of space-based Earth observation relevant to the study of large river systems through the provision of spatially continuous, synoptic and temporally repetitive multi-spectral data. Free access to the Landsat archive from mid-2008 has enabled the scientific community to reconstruct the Earth’s changing surface and, in particular, to reconstruct the planform dynamics of the world’s largest rivers. -
A Reduced Complexity Model of a Gravel-Sand River Bifurcation: Equilibrium States and Their Stability
Advances in Water Resources 121 (2018) 9–21 Contents lists available at ScienceDirect Advances in Water Resources journal homepage: www.elsevier.com/locate/advwatres A reduced complexity model of a gravel-sand river bifurcation: Equilibrium states and their stability Ralph M.J. Schielen a,b,∗, Astrid Blom c a Faculty of Engineering Technology, University of Twente, Netherlands b Ministry of Infrastructure and Water Management-Rijkswaterstaat, Netherlands c Faculty of Civil Engineering and Geosciences, Delft University of Technology, Netherlands a r t i c l e i n f o a b s t r a c t Keywords: We derive an idealized model of a gravel-sand river bifurcation and analyze its stability properties. The model River bifurcation requires nodal point relations that describe the ratio of the supply of gravel and sand to the two downstream Mixed-size sediment branches. The model predicts changes in bed elevation and bed surface gravel content in the two bifurcates Idealized model under conditions of a constant water discharge, sediment supply, base level, and channel width and under the Equilibrium assumption of a branch-averaged approach of the bifurcates. The stability analysis reveals more complex behavior Stability analysis than for unisize sediment: three to five equilibrium solutions exist rather than three. In addition, we find that under specific parameter settings the initial conditions in the bifurcates determine to which of the equilibrium states the system evolves. Our approach has limited predictive value for real bifurcations due to neglecting several effects (e.g., transverse bed slope, alternate bars, upstream flow asymmetry, and bend sorting), yet it provides a first step in addressing mixed-size sediment mechanisms in modeling the dynamics of river bifurcations. -
Partitioning of Water and Sediment Over Bifurcation Points Under the Influence of Climate Change: Consequences and Mitigation
Partitioning of water and sediment over bifurcation points under the influence of climate change: consequences and mitigation NKWK RIVERS2MORROW @Twente University Team • Supervisors: Ralph Schielen(PI, UT/RWS), Denie Augustijn (UT), Maarten Kleinhans (UU), Astrid Blom (TUD) • Proposed members Advisory Group: Jord Warmink (UT), Pepijn van Denderen (UT), Max Schropp, (RWS), Mohammed Yossef (Deltares), Marcello Garcia (Illinois) Introduction When lowland rivers approach the sea, they form deltas that are characterized by the presence of bifurcation points. In pristine circumstances, a delta is dynamic and changes constantly due to avulsions. As a result, a delta is characterized by (many) bifurcation points where the river splits into two (or more) branches which eventually builds the characteristic form of a delta. It is a dynamic pattern of branches. In engineered rivers, bifurcation points exist within confined geometry. The confinement comes from the presence of levees, groynes and revetments. It depends on the location of the bifurcation point (upper delta, lower delta) whether gravel, sand, silt or a gravel-sand or sand-silt mixture is distributed over the branches. Examples of bifurcation points in engineered rivers can be found in, among others, the Netherlands (the Rhine river), in Romania (the Lower Danube), in the USA (Mississippi) and in Myanmar (the Ayeyarwady river) (see figure 1). Stability of river bifurcations is important for safety reasons and for navigation. Stability in this context means, that the bifurcating branch stays open (does not silt up) and hence, that the distribution of water and sediment remains between reasonable bounds. In the Netherlands for instance, the safety downstream of the bifurcation points in the Rhine River is determined under the condition of a fixed partition of the discharge over the bifurcation points (Schielen et al. -
REYNOLDS, JENNIFER L., MA Morphological Response To
REYNOLDS, JENNIFER L., M.A. Morphological Response to Reduced Discharge on a Losing Catawba River Bifurcate. (2016) Directed by Dr. Dan Royall. 74 pp. River Channel bifurcations resulting from partial avulsions are features of fluvial systems that remain poorly understood. The southeastern Piedmont of North Carolina is an area where large bifurcated rivers are uncommon, yet in an area near the foot of the Blue Ridge Escarpment several prominent contemporary examples exist. The initiation of these uncommon bifurcations and the subsequent persistence of split flow in these river reaches (Catawba, Linville, Yadkin Rivers and Wilson Creek) are yet to be fully understood. This study entailed GIS spatiotemporal analysis of planform morphologies, hydraulic geometry and geomorphic analysis of river bank sedimentation and channel narrowing in the losing branch of a prominent Catawba River bifurcation, that are believed to influence the long-term stability of bifurcated channel patterns, and allows for determining pre and post bifurcation states, morphostratigraphic surveys of channel bank deposits, the nature of sedimentation events contributing most to channel adjustments over the last 39 years. It is important to study and understand the evolution of river bifurcations and the processes of avulsion that produce them because of the significant implications of these events pertaining to infrastructure management (roads, bridges and dwellings), flood hazard assessment and zonation, land conservation, as well as riverine ecosystems. MORPHOLOGICAL RESPONSE TO REDUCED DISCHARGE ON A LOSING CATAWBA RIVER BIFURCATE by Jennifer L. Reynolds A Thesis Submitted to the Faculty of The Graduate School at The University of North Carolina at Greensboro in Partial Fulfillment of the Requirements for the Degree Master of Arts Greensboro 2016 Approved by ____Dr. -
APC&EC Regulation 2
;.. : . : ., (L 1,.1 '"~ · .;n. Pollution Control and Ecology corrimiSsi 1¥# eJ14.€JC-0(91.l - .. ·~. ·~ .... ARKANSAS POLLUTION CONTROL AND ECOLOGY COMMISSION REGULATION NO.2 REGULATION ESTABLISHING WATER QUALITY STANDARDS FOR SURFACE WATERS OF THE STATE OF ARKANSAS Adopted by the Arkansas Pollution Control and Ecology Commission: December 7, 2018 Arkansas Pollution Control and Ecology Commission Regulation No. 2, As Amended Regulation Establishing Water Quality Standards for Surface Waters of the State of Arkansas TABLE OF CONTENTS CHAPTER 1: AUTHORITY, GENERAL PRINCIPLES, AND COVERAGE ........................ 1-1 Reg. 2.101 Authority .............................................................................................. 1-1 Reg. 2.102 Purpose ................................................................................................. 1-1 Reg. 2.103 Commission Review ............................................................................ 1-2 Reg. 2.104 Policy for Compliance .......................................................................... 1-2 Reg. 2.105 Environmental Improvement Projects .................................................. 1-2 Reg. 2.106 Definitions ............................................................................................ 1-2 CHAPTER 2: ANTIDEGRADATION POLICY ....................................................................... 2-1 Reg. 2.201 Existing Uses ........................................................................................ 2-1 Reg. 2.202 High Quality -
Predicting Water and Sediment Partitioning in a Delta Channel
RESEARCH ARTICLE Predicting Water and Sediment Partitioning in a Delta 10.1029/2020WR027199 Channel Network Under Varying Key Points: • Channel width, sinuosity, and Discharge Conditions bifurcation angle are the best Tian Y. Dong1 , Jeffrey A. Nittrouer1 , Brandon McElroy2 , Elena Il'icheva3,4, remotely sensed, nodal relation 3 1 1 5 variables for predicting flow Maksim Pavlov , Hongbo Ma , Andrew J. Moodie , and Vsevolod M. Moreido partitioning 1 2 • Channel cross‐sectional area and Department of Earth, Environmental, and Planetary Sciences, Rice University, Houston, TX, USA, Department of flow depth are the best field Geology and Geophysics, University of Wyoming, Laramie, WY, USA, 3Laboratory of Hydrology and Climatology, V.B. measured, nodal relation variables Sochava Institute of Geography, Siberian Branch Russian Academy of Sciences, Irkutsk, Russia, 4Department of for predicting flow partitioning Hydrology and Environmental Sciences, Irkutsk State University, Irkutsk, Russia, 5Water Problems Institute, Russian • A field validated graph model is developed to accurately predict flux Academy of Sciences, Moscow, Russia distribution in deltas, where gauge data are limited Abstract Channel bifurcations control the distribution of water and sediment in deltas, and the routing Supporting Information: of these materials facilitates land building in coastal regions. Yet few practical methods exist to provide • Supporting Information S1 accurate predictions of flow partitioning at multiple bifurcations within a distributary channel network. Herein, multiple nodal relations that predict flow partitioning at individual bifurcations, utilizing various hydraulic and channel planform parameters, are tested against field data collected from the Selenga River Correspondence to: ‐ T. Y. Dong, delta, Russia. The data set includes 2.5 months of time continuous, synoptic measurements of water and [email protected] sediment discharge partitioning covering a flood hydrograph.