High Voltage Subnanosecond Dielectric Breakdown John
Total Page:16
File Type:pdf, Size:1020Kb
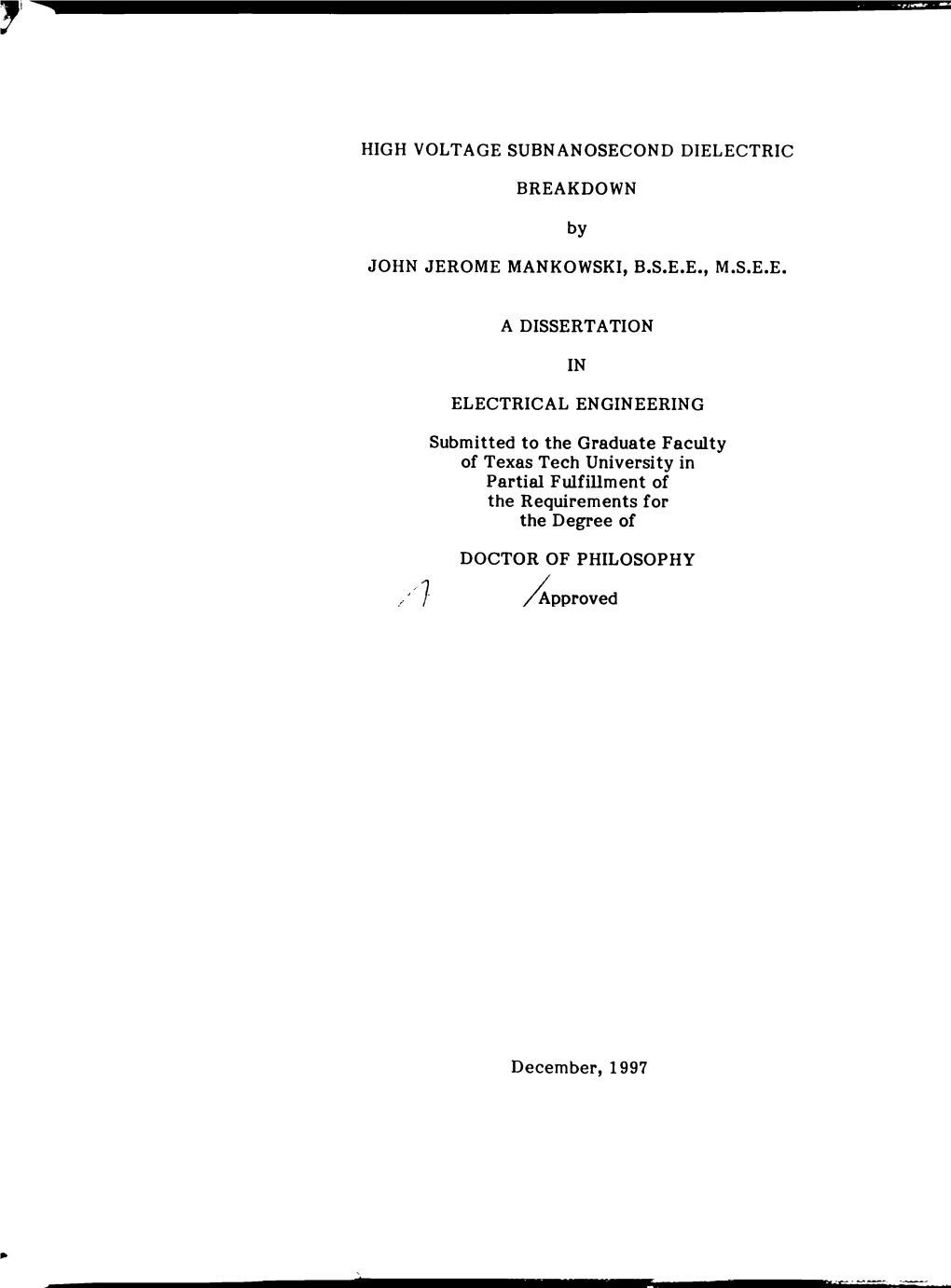
Load more
Recommended publications
-
Methodology for Analysis of Electrical Breakdown in Micrometer Gaps In
Methodology for Analysis of Electrical Breakdown In Micrometer gaps in Tip-To-Plane Configuration Kemas Tofani, Jean-Pascal Cambronne, Sorin Dinculescu, Ngapuli Sinisuka, Kremena Makasheva To cite this version: Kemas Tofani, Jean-Pascal Cambronne, Sorin Dinculescu, Ngapuli Sinisuka, Kremena Makasheva. Methodology for Analysis of Electrical Breakdown In Micrometer gaps in Tip-To-Plane Configuration. 2018 IEEE 13th Nanotechnology Materials and Devices Conference (NMDC), Oct 2018, Portland, United States. pp.1-4, 10.1109/NMDC.2018.8605855. hal-02324370 HAL Id: hal-02324370 https://hal.archives-ouvertes.fr/hal-02324370 Submitted on 1 Nov 2019 HAL is a multi-disciplinary open access L’archive ouverte pluridisciplinaire HAL, est archive for the deposit and dissemination of sci- destinée au dépôt et à la diffusion de documents entific research documents, whether they are pub- scientifiques de niveau recherche, publiés ou non, lished or not. The documents may come from émanant des établissements d’enseignement et de teaching and research institutions in France or recherche français ou étrangers, des laboratoires abroad, or from public or private research centers. publics ou privés. Methodology for analysis of electrical breakdown in micrometer gaps in tip-to-plane configuration Kemas M Tofani LAPLACE, Université de Toulouse, Jean-Pascal Cambronne Sorin Dinculescu CNRS LAPLACE, Université de Toulouse, LAPLACE, Université de Toulouse, Toulouse, France CNRS CNRS Bandung Institute of Technology Toulouse, France Toulouse, France Bandung, Indonesia [email protected] [email protected] PT. PLN Jakarta, Indonesia Kremena Makasheva [email protected] LAPLACE, Université de Toulouse, CNRS Ngapuli I. Sinisuka Toulouse, France Bandung Institute of Technology [email protected] Bandung, Indonesia [email protected] Abstract—Better understanding of the electrical behavior of electron emission process by ions bombardment on the miniaturized electrical devices is largely supported by the need cathode. -
The Pennsylvania State University
The Pennsylvania State University The Graduate School Department of Materials Science and Engineering TEMPERATURE DEPENDENCE OF DIELECTRIC BREAKDOWN IN POLYMERS A Thesis in Materials Science and Engineering by Cheolhong Min © 2008 Cheolhong Min Submitted in Partial Fulfillment of the Requirements for the Degree of Master of Science August 2008 ii The thesis of Cheolhong Min was reviewed and approved* by the following: Thomas R. Shrout Professor of Materials Science Thesis Co-Advisor Michael T. Lanagan Associate Professor of Engineering Science and Mechanics Thesis Co-Advisor Shujun Zhang Research Associate and Assistant Professor of Materials Science and Engineering Joan Redwing Professor of Materials Science and Engineering Chair, Intercollege Graduate Degree Program in Materials Science and Engineering *Signatures are on file in the Graduate School iii ABSTRACT Capacitors possess high-power densities and have the ability to deliver energy with short discharge times, which are in the micro-second to nano-second range. Both energy and power density are related to the dielectric materials used in the capacitor. One of the main challenges for capacitors is achieving high energy density as determined by the relative permittivity and dielectric breakdown strength of a material. Polymers are some of the most important dielectric materials for high-power capacitors because polymer films show high breakdown strength. A general trend in polymers is that the breakdown strength increases with decreasing temperature. An understanding of the temperature dependence relationships among electrical properties, polymer chemistry, and crystalline structure may lead to improved energy storage for polymer-based capacitors—this is the basis of the thesis. Various polymers including polypropylene (PP), polyimide (PI), polymethyl methacrylate (PMMA), poly(vinylidene fluoride-trifluoroethylene- chlorotrifluoroethylene) terpolymer (p(VDF-TrFE-CTFE)) were investigated in this study. -
Liquid Dielectrics, Their Classification, Properties, and Breakdown Strength
CHAPTER 6 LIQUID DIELECTRICS, THEIR CLASSIFICATION, PROPERTIES, AND BREAKDOWN STRENGTH From the point of view of molecular arrangement, a liquid can be described as “ highly compressed gas ” in which the molecules are very closely arranged. This is known as kinetic model of the liquid structure. A liquid is characterized by free movement of the constituent molecules among themselves but without the tendency to separate. However, the movement of charged particles, their microscopic streams, and interface conditions with other materials cause a distortion in the otherwise undisturbed molecular structure of the liquids. The well known terminology describ- ing the breakdown mechanisms in gaseous dielectrics — such as impact ionization, mean free path, electron drift, and so on — are, therefore, also applicable for liquid dielectrics. Liquid dielectrics are accordingly classifi ed in between the two states of matter; that is, gaseous and solid insulating materials. Wide range of application of liquid dielectircs in power apparatus also characterizes this intermediate position of liquid dielectrics. Insulating oils are used in power and instrument transformers, power cables, circuit breakers, power capacitors, and so on. Liquid dielectrics perform a number of functions simultaneously, namely: • insulation between the parts carrying voltage and the grounded container, as in transformers • impregnation of insulation provided in thin layers of paper or other materials, as in transformers, cables and capacitors, where oils or impregnating com- pounds are used • cooling action by convection in transformers and oil fi lled cables through circulation • fi lling up of the voids to form an electrically stronger integral part of a com- posite dielectric • arc extinction in circuit breakers High Voltage and Electrical Insulation Engineering, First Edition. -
Chapter Iii Power Bipolar Junction Transistor (Bjt)
50 CHAPTER III POWER BIPOLAR JUNCTION TRANSISTOR (BJT) 3.1 INTRODUCTION Switching time and switching losses are primary concerns in high power applications. These two factors can significantly influence the frequency of operation and the efficiency of the circuit. Ideally, a high power switch should be able to turn-on and turn-off controllably and with minimum switching loss. The Bipolar junction transistor is an important power semiconductor device used as a switch in a wide variety of applications. The switching speed of a BJT is often limited by the excess minority charge storage in the base and collector regions of the transistor during the saturation state. The conventional methods for improving the switching frequency by reducing the lifetime of the lightly doped collector region through incorporation of impurities such as Au, Pt or by introducing radiation-induced defects have been found unsuitable for high voltage devices due to increased leakage, soft breakdown and high ‘ON’ state voltage [27]. Among the techniques proposed to overcome these problems, use of universal contact (UC) [2, 14] is particularly promising. The present work looks in detail at the various aspects arising out of incorporation of UC in BJTs. The UC is incorporated in the transistor by creating additional diffused regions in an otherwise conventional transistor. These diffused regions influence the minority carrier distribution, nature of minority current flow and also some other parameter such as VCE(sat). To study these phenomena, 51 an analytical model is developed and is utilized to understand the effect of universal contact on reverse recovery, VCE(sat) and other related issues. -
“Contribution to Liquid Lens Technology”
“CONTRIBUTION TO LIQUID LENS TECHNOLOGY” Thesis submitted in partial fulfillment of the requirement for the PhD Degree issued by the Universitat Politècnica de Catalunya, in its Electronic Engineering Program Nom del doctorand Maziar Ahmadi Zeidabadi Directors: Prof. Dr. Luis Castañer Prof. Dra. Sandra Bermejo data de lectura de la tesi Mayo 2016 2 3 4 Contents Contents .............................................................................................................................. 5 1 Summary ................................................................................................................... 21 1.1 Introduction........................................................................................................ 23 1.2 Thesis challenges and outlines .......................................................................... 25 2 Liquid lens (Fundamentals and theory) ................................................................... 27 2.1 Liquid lens devices overview ............................................................................ 29 2.2 Basic principles of capillarity and wetting ........................................................ 30 2.3 Hydrophobic surfaces ........................................................................................ 32 2.3.1 Wenzel model ............................................................................................. 33 2.3.2 Cassie-Baxter model................................................................................... 34 2.4 Electrical -
Download Technical Paper
TECHNICAL PAPER Thermal and Electrical Breakdown Versus Reliability of Ta2O5 under Both – Bipolar Biasing Conditions P. Vašina, T. Zedníček, Z. Sita AVX Czech Republic, s.r.o., Dvorakova 328, 563 01 Lanskroun, Czech Republic J. Sikula and J. Pavelka CNRL TU Brno, Technicka 8, 602 00 Brno, Czech Republic Abstract: Our investigation of breakdown is mainly oriented to find a basic parameters describing the phenomena as well as its impact on reliability and quality of the final product that is “GOOD” tantalum capacitor. Basically, breakdown can be produced by a number of successive processes: thermal breakdown because of increasing conductance by Joule heating, avalanche and field emission break, an electromechanical collapse due to the attractive forces between electrodes electrochemical deterioration, dendrite formation and so on. Breakdown causes destruction in the insulator and across the electrodes mainly by melting and evaporation, sometimes followed by ignition. An identification of breakdown nature can be achieved from VA characteristics. Therefore, we have investigated the operating parameters both in the normal mode, Ta is a positive electrode, as well as in the reverse mode with Ta as a negative one. In the reverse mode we have reported that the thermal breakdown is initiated by an increase of the electrical conductance by Joule heating. Consequently followed in a feedback cycle consisting of temperature - conductivity - current - Joule heat - temperature. In normal mode an electrical breakdown can be stimulated by an increase of the electrical conductance in a channel by an electrical pulse and stored charge leads to the sample destruction. Both of these breakdowns have got a stochastic behaviour and could be hardly localized in advance. -
ECE 255, Diodes and Rectifiers
ECE 255, Diodes and Rectifiers 23 January 2018 In this lecture, we will discuss the use of Zener diode as voltage regulators, diode as rectifiers, and as clamping circuits. 1 Zener Diodes In the reverse biased operation, a Zener diode displays a voltage breakdown where the current rapidly increases within a small range of voltage change. This property can be used to limit the voltage within a small range for a large range of current. The symbol for a Zener diode is shown in Figure 1. Figure 1: The symbol for a Zener diode under reverse biased (Courtesy of Sedra and Smith). Figure 2 shows the i-v relation of a Zener diode near its operating point where the diode is in the breakdown regime. The beginning of the breakdown point is labeled by the current IZK also called the knee current. The oper- ating point can be approximated by an incremental resistance, or dynamic resistance described by the reciprocal of the slope of the point. Since the slope, dI proportional to dV , is large, the incremental resistance is small, generally on the order of a few ohms to a few tens of ohms. The spec sheet usually gives the voltage of the diode at a specified test current IZT . Printed on March 14, 2018 at 10 : 29: W.C. Chew and S.K. Gupta. 1 Figure 2: The i-v characteristic of a Zener diode at its operating point Q (Cour- tesy of Sedra and Smith). The diode can be fabricated to have breakdown voltage of a few volts to a few hundred volts. -
Capacitive Power Transfer Through Rotational and Sliding Bearings
CAPACITIVE POWER TRANSFER THROUGH ROTATIONAL AND SLIDING BEARINGS by Skyler S. Hagen A thesis submitted in partial fulfillment of the requirements for the degree of: Master of Science (Electrical Engineering) at the UNIVERSITY OF WISCONSIN – MADISON 2016 i Abstract Throughout the history of electrification, applications have existed for the transmission of electrical energy from stationary sources to moving loads. Electrical equipment which is expected to move along tracks, or in cyclical, pivoting, or rotational patterns of motion often requires externally-supplied electrical power to operate. Various techniques have been used with success in the past such as brushes with sliding contacts [1], cable connections (when practical), and various inductive and capacitive contactless power transfer strategies [2],[3],[4],[5]; however, each has its own limitations in longevity and/or complexity. Applications for power transfer to moving loads proliferate in the automotive and traction industries, as well as automation and manufacturing. Both of these categories have strict requirements on reliability. Failure in operation can be hazardous to human life and property in the case of transportation and heavy equipment traction. In the case of manufacturing, the reliability requirement is justified by the large opportunity cost incurred by machine down time. The following is a proposition for a technology which is well suited for many key modern applications. Using the nanofarad-scale capacitance already present in a variety of rotational and linear journal bearings, along with a simple soft-switching high frequency power converter circuit, power levels in the 102-103 watt range have successfully been transferred capacitively from stationary power sources to moving loads. -
Preparation of Monodisperse Microbubbles in a Capillary Embedded T-Junction Device and the Influence of Process Control Parameters on Bubble Size and Stability
Preparation of monodisperse microbubbles in a capillary embedded T-Junction device and the influence of process control parameters on bubble size and stability A thesis submitted in partial fulfilment of the requirements for the degree of Doctor of Philosophy By Maryam Parhizkar Department of Mechanical Engineering University College London Torrington Place, London WC1E 7JE U.K March, 2014 Declaration I, Maryam Parhizkar, confirm that the work presented in this thesis is my own. Where information has been derived from other sources, I confirm that this has been indicated in the thesis. ………………………………….. Maryam Parhizkar 1 Abstract The main goal for this work was to produce microbubbles for a wide range of applications with sizes ranging between 10 to 300 µm in a capillary embedded T- junction device. Initially the bubble formation process was characterized and the factors that affected the bubble size; in particular the parameters that reduce it were determined. In this work, a polydimethylsiloxane (PDMS) block (100 x 100 x 10 mm3) was used, in which the T-shaped junction was created by embedded capillaries of fixed outer diameter. The effect of the inner diameter was investigated by varying all the inlet and outlet capillaries’ inner diameter at different stages. In addition, the effect of changes in the continuous phase viscosity and flow rate (Ql) as well as the gas pressure (Pg) on the resulting bubble size was studied. Aqueous glycerol solutions were chosen for the liquid phase, as they are widely used in experimental studies of flow phenomena and provide a simple method of varying properties through dilution. -
Capacitors and Dielectrics Dielectric - a Non-Conducting Material (Glass, Paper, Rubber….)
Capacitors and Dielectrics Dielectric - A non-conducting material (glass, paper, rubber….) Placing a dielectric material between the plates of a capacitor serves three functions: 1. Maintains a small separation between the plates 2. Increases the maximum operating voltage between the plates. 3. Increases the capacitance In order to prove why the maximum operating voltage and capacitance increases we must look at an atomic description of the dielectric. For now we will show that the capacitance increases by looking at an experimental result: Experiment Since VVCCoo, then Since Q is the same: Coo V CV C V o K CVo C K Dielectric Constant Co Since C > Co , then K > 1 C kCo V V o k kvacuum = 1 (vacuum) k = 1.00059 (air) kglass = 5-10 Material Dielectric Constant k Dielectric Strength (V/m) Air 1.00054 3 Paper 3.5 16 Pyrex glass 4.7 14 A real dielectric is not a perfect insulator and thus you will always have some leakage current between the plates of the capacitor. For a parallel-plate capacitor: C kCo kA C o d From this equation it appears that C can be made as large as possible by decreasing d and thus be able to store a very large amount of charge or equivalently a very large amount of energy. Is there a limit on how much charge (energy) a capacitor can store? YES!! +q K e- - F =qE E e e- E dielectric -q As charge ‘q’ is added to the capacitor plates, the E-field will increase until the dielectric becomes a conductor (dielectric breakdown). -
Power MOSFET Basics by Vrej Barkhordarian, International Rectifier, El Segundo, Ca
Power MOSFET Basics By Vrej Barkhordarian, International Rectifier, El Segundo, Ca. Breakdown Voltage......................................... 5 On-resistance.................................................. 6 Transconductance............................................ 6 Threshold Voltage........................................... 7 Diode Forward Voltage.................................. 7 Power Dissipation........................................... 7 Dynamic Characteristics................................ 8 Gate Charge.................................................... 10 dV/dt Capability............................................... 11 www.irf.com Power MOSFET Basics Vrej Barkhordarian, International Rectifier, El Segundo, Ca. Discrete power MOSFETs Source Field Gate Gate Drain employ semiconductor Contact Oxide Oxide Metallization Contact processing techniques that are similar to those of today's VLSI circuits, although the device geometry, voltage and current n* Drain levels are significantly different n* Source t from the design used in VLSI ox devices. The metal oxide semiconductor field effect p-Substrate transistor (MOSFET) is based on the original field-effect Channel l transistor introduced in the 70s. Figure 1 shows the device schematic, transfer (a) characteristics and device symbol for a MOSFET. The ID invention of the power MOSFET was partly driven by the limitations of bipolar power junction transistors (BJTs) which, until recently, was the device of choice in power electronics applications. 0 0 V V Although it is not possible to T GS define absolutely the operating (b) boundaries of a power device, we will loosely refer to the I power device as any device D that can switch at least 1A. D The bipolar power transistor is a current controlled device. A SB (Channel or Substrate) large base drive current as G high as one-fifth of the collector current is required to S keep the device in the ON (c) state. Figure 1. Power MOSFET (a) Schematic, (b) Transfer Characteristics, (c) Also, higher reverse base drive Device Symbol. -
Electrical Breakdown in Vacuum 1
ELECTRICAL B RE AKDOWN IN VACUUM G.P. BEUKEMA Stellingen behorende bij het prdefschrift ELECTRICAL BREAKDOWN IN VACUUM 1. De door Coolen en Van Schaik opgemeten profielen van de D2~absorptielijn van z3Na in neon worden bepaald door een combinatie van de overgangswaar- schijnlijkheden tussen de niveaus en van het laservermogen. Zij hadden een uitspraak kunnen doen over de invloed van beide effekten als zij de profielen niet alleen bij verschillende laservermogens hadden opgemeten, maar ook bij verschillende dichtheden van het neongas. F.C.M. Coolen en N. van Schaik, Physica 93 C, (1978) 261 - 6. 2. De uitspraak, gedaan in "Structure of Physics" door G.R. Noakes, dat inter- ferentie van fotonen bij de proef van Young plaatsvindt nadat deze de twee spleten gepasseerd zijn, of dat een bepaald foton geen wisselwerking heeft met elk van de spleten, is onjuist. • G.R. Noakes, Structure of Physics, Mac Millan (1977), blz. 468 en 502. 3. Voor het waarnemen van het ontstaan en de groei van interne disrupties in Tokamak plasma's verdienen cyclotron-stralingsmetingen de voorkeur boven röntgen-metingen. 4. De door Knudson en Nimrod voorgestelde exacte vergelijking voor de bereke- ning van titratiecurven voor twee-basische zouten is niet exact. G.E. Knudson en D. Nimrod, J. Chem. Educ. _54_ (1977) 351. 5. Plasma's kunnen de 5 aggregatietoestand van de materie genoemd worden, niet de 4 , zoals te doen gebruikelijk is. 6. De term "desorption cross section", zoals ingevoerd door Taglauer et al. kan aanleiding geven tot misverstand. E. Taglauer, U. Beitat, G. Marin en W. Heiland, J.