Shear-Wave Splitting and Attenuation Analysis of Downhole Microseismic Data for Reservoir Characterization of the Montney Formation, Pouce Coupe, Alberta
Total Page:16
File Type:pdf, Size:1020Kb
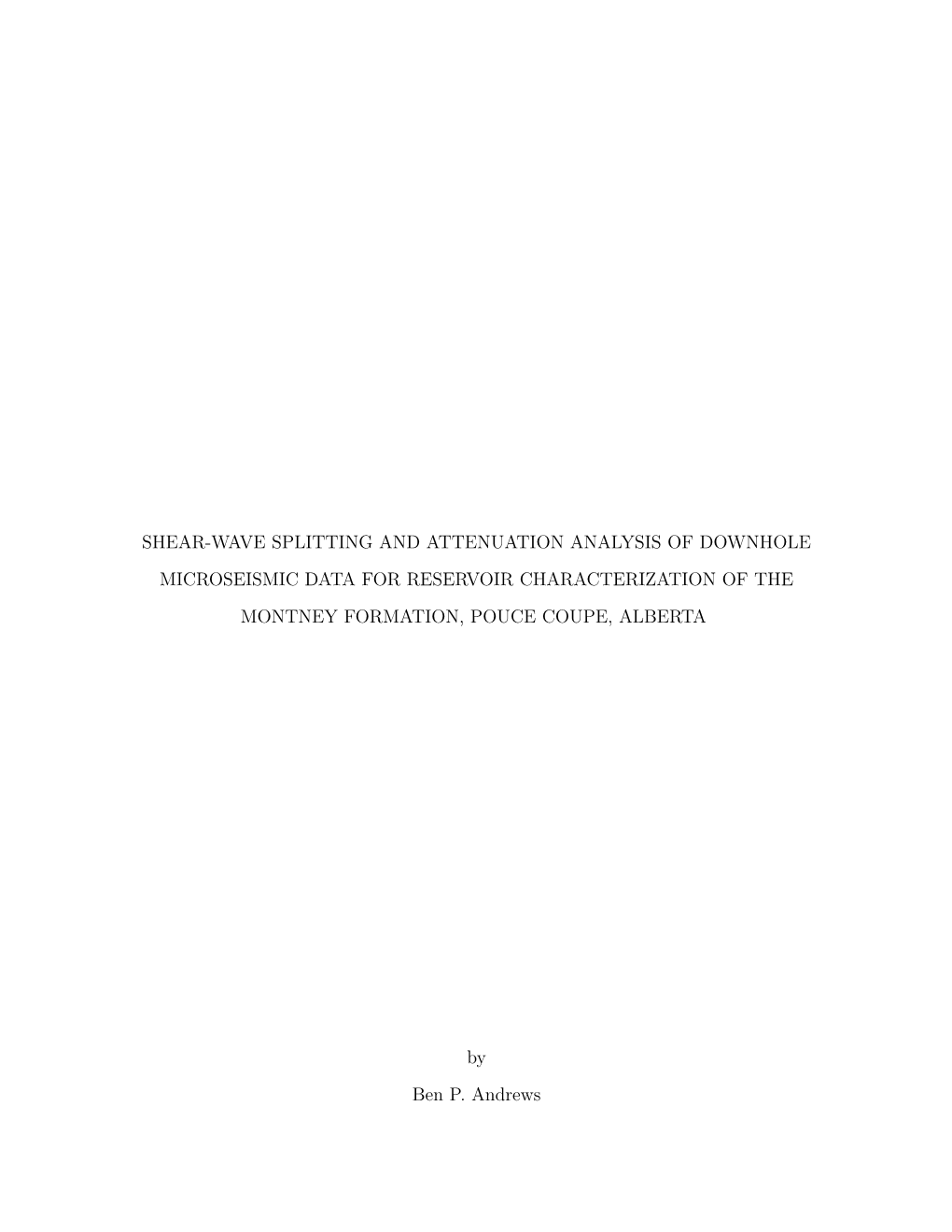
Load more
Recommended publications
-
Shear Wave Splitting Time Variation by Stress-Induced Magma Uprising at Mount Etna Volcano
Shear wave splitting time variation by stress-induced magma uprising at Mount Etna volcano Francesca Bianco1, Luciano Scarfì2, Edoardo Del Pezzo1 & Domenico Patanè2 1Istituto Nazionale Geofisica e Vulcanologia - Osservatorio Vesuviano, Napoli (Italy) 2 Istituto Nazionale Geofisica e Vulcanologia Sez. Catania (Italy) Abstract Shear wave splitting exhibits clear time variations before the July 17th – August 9th, 2001 flank eruption at Mount Etna. The normalized time delays, Tn, detected through an orthogonal transformation of singular value decomposition, exhibit a clear increase starting 20 days before the occurrence of the eruption (July 17th); the qS1 polarization direction, obtained using a 3D covariance matrix decomposition, shows a 90°-flip several times during the analyzed period: the last flip 5 days before the occurrence of the eruption. Both splitting parameters also exhibit a relaxation phase shortly before the starting of the eruption. Our observations seem in agreement with Anisotropic Poro Elasticity (APE) modelling, suggesting a tool for the temporal monitoring of the build up of the stress leading to the occurrence of the 2001 eruption at Mt. Etna. Introduction Shear wave splitting is the elastic analogue of the well known optical birefringence phenomenon. A shear wave entering into an anisotropic volume, is split into two quasi shear waves, qS1 and qS2, propagating with different velocities and approximately orthogonal polarizations. The splitting parameters are the time delay between qS1 and qS2 wave (hereafter referred to as Td); and the polarization direction of the leading split (qS1) wave (hereafter referred to as Lspd, Linear split polarization direction).. The Extensive Dilatancy Anisotropy (EDA) model[Crampin et al, 1984], suggests that the principal source of seismic anisotropy in the crust is stress aligned fluid filled microcracks. -
Seismic Anisotropy and Shear- Wave Splitting
Seismic Anisotropy and shear- wave splitting Steve Gao, Missouri S&T http://www.mst.edu/~sgao 1 Anisotropy and Seismic Anisotropy • Anisotropy – property of a material is directionally dependent • Seismic anisotropy – seismic property (i.e., traveling velocity of waves) is directionally dependent 2 What is seismic anisotropy? • For P-waves and same-type surface waves: dependence of seismic wave speed on the direction of propagation of the waves • For S-waves and different type surface waves: dependence of seismic wave speed on the polarization (or vibration) direction of the waves 3 Seismic Phases Inside the earth, the velocity is not a smooth function of depth. To make things more complicated, there are several major interfaces including the core-mantle boundary and the outer core-inner core boundary, as well as the crust- mantle boundary (Moho). At the boundaries (and also at everywhere), the raypath is controlled by the Snell’s law Q: Why the raypath curves toward the center in the outer core? What kind of wave is it? 4 Raypaths for selected seismic waves P: direct P wave traveled (refracted) through the mantle PP: P wave refracted from the mantle, reflected at Earth’s surface,then refracted again PPP: P wave reflected twice at the Earth’s surface PKP: P wave refracted from the mantle through the outer core, then back through the mantle PKIKP: P wave refracted from the mantle, through the outer core, inner core and back out PKiKP: P wave bounced back from the inner core/outer core boundary PcP: P wave reflected off the outer core 5 -
Faults (Shear Zones) in the Earth's Mantle
Tectonophysics 558-559 (2012) 1–27 Contents lists available at SciVerse ScienceDirect Tectonophysics journal homepage: www.elsevier.com/locate/tecto Review Article Faults (shear zones) in the Earth's mantle Alain Vauchez ⁎, Andréa Tommasi, David Mainprice Geosciences Montpellier, CNRS & Univ. Montpellier 2, Univ. Montpellier 2, cc. 60, Pl. E. Bataillon, F-34095 Montpellier cedex5, France article info abstract Article history: Geodetic data support a short-term continental deformation localized in faults bounding lithospheric blocks. Received 23 April 2011 Whether major “faults” observed at the surface affect the lithospheric mantle and, if so, how strain is distrib- Received in revised form 3 May 2012 uted are major issues for understanding the mechanical behavior of lithospheric plates. A variety of evidence, Accepted 3 June 2012 from direct observations of deformed peridotites in orogenic massifs, ophiolites, and mantle xenoliths to seis- Available online 15 June 2012 mic reflectors and seismic anisotropy beneath major fault zones, consistently supports prolongation of major faults into the lithospheric mantle. This review highlights that many aspects of the lithospheric mantle defor- Keywords: Faults/shear-zones mation remain however poorly understood. Coupling between deformation in frictional faults in the upper- Lithospheric mantle most crust and localized shearing in the ductile crust and mantle is required to explain the post-seismic Field observations deformation, but mantle viscosities deduced from geodetic data and extrapolated from laboratory experi- Seismic reflection and anisotropy ments are only reconciled if temperatures in the shallow lithospheric mantle are high (>800 °C at the Rheology Moho). Seismic anisotropy, especially shear wave splitting, provides strong evidence for coherent deforma- Strain localization tion over domains several tens of km wide in the lithospheric mantle beneath major transcurrent faults. -
The Hawaii-2 Observatory: Observation of Nanoearthquakes, Seismol
Butler, R. The Hawaii-2 Observatory: Observation of Nanoearthquakes, Seismol. Res. Lett., 74(10), 290-297, (2003). 1 The Hawaii-2 Observatory: Observation of Nanoearthquakes Rhett Butler The IRIS Consortium, Washington DC Introduction The Hawaii-2 Observatory (H2O, http://www.iris.edu/GSN/) was established on the seafloor between Hawaii and California (Figure 1) in 1998 using the retired AT&T Hawaii-2 undersea telephone cable for power and telemetry (Butler et al., 2000; Duennebier et al., 2002, Pettit et al., 2002; Harris and Duennebier, 2002). This is the first undersea seismic station in the Global Seismographic Network. The on-site instrumentation includes a broadband Guralp CMG-3 and 4.5 Hz Geospace HS1 geophones, buried 0.5 m into the seafloor mud, and an OAS E2PD hydrophone 0.5 m above the seafloor. All of the data are natively sampled at 160 sps continuously and sent via the Hawaii-2 cable to Oahu where the data are distributed by the University of Hawaii to IRIS. Data are available in real-time via a Live Internet Seismic Server (LISS) server, and are being used by the Pacific Tsunami Warning Center and the National Seismic Network. In early 2002, the Ocean Drilling Program drilled a borehole through 29±1 m of sediment into basalt about 1.5 km northeast of the current H2O instrumentation as a site for a future borehole sensor (Stephen et al., 2003). H2O was primarily sited to fill a large gap in global coverage between Hawaii and California, and not to monitor any particular local or regional seismicity. -
Isotropic and Anisotropic P and S Velocities of the Baltic Shield Mantle
Digital Comprehensive Summaries of Uppsala Dissertations from the Faculty of Science and Technology 653 Isotropic and Anisotropic P and S Velocities of the Baltic Shield Mantle Results from Analyses of Teleseismic Body Waves TUNA EKEN ACTA UNIVERSITATIS UPSALIENSIS ISSN 1651-6214 UPPSALA ISBN 978-91-554-7548-2 urn:nbn:se:uu:diva-102501 2009 Dissertation presented at Uppsala University to be publicly examined in Hambergsalen, Geocentrum, Villavägen 16, SE-752 36 Uppsala, Sweden, Uppsala, Friday, June 12, 2009 at 13:00 for the degree of Doctor of Philosophy. The examination will be conducted in English. Abstract Eken, T. 2009. Isotropic and Anisotropic P and S Velocities of the Baltic Shield Mantle. Results from Analyses of Teleseismic Body Waves. Acta Universitatis Upsaliensis. Digital Comprehensive Summaries of Uppsala Dissertations from the Faculty of Science and Technology 653. 110 pp. Uppsala. ISBN 978-91-554-7548-2. The upper mantle structure of Swedish part of Baltic Shield with its isotropic and anisotropic seismic velocity characteristics is investigated using telesesismic body waves (i.e. P waves and shear waves) recorded by the Swedish National Seismological Network (SNSN). Nonlinear high-resolution P and SV and SH wave isotropic tomographic inversions reveal velocity perturbations of ± 3 % down to at least 470 km below the network. Separate SV and SV models indicate several consistent major features, many of which are also consistent with P- wave results. A direct cell by cell comparison of SH and SV models reveals velocity differences of up to 4%. Numerical tests show that differences in the two S-wave models can only be partially caused by noise and limited resolution, and some features are attributed to the effect of large scale anisotropy. -
Magma-Assisted Rifting in Ethiopia
NATURE 3161—7/12/2004—VBICKNELL—128523 letters to nature .............................................................. shear waves (dt) and the orientation of the fast shear wave (f). To Magma-assisted rifting in Ethiopia remove the effects of the anisotropy one can rotate the horizontal components by f and shift their relative positions by dt, thereby linearizing the particle motion and removing the transverse J.-M. Kendall1, G. W. Stuart1, C. J. Ebinger2, I. D. Bastow1 & D. Keir2 component energy on the seismograms10,11. To estimate the splitting 1School of Earth Sciences, University of Leeds, Leeds LS2 9JT, UK we search for the correction parameters that best linearize the SKS 2Department of Geology, Royal Holloway, University of London, Egham, motion (that is, minimize the smaller eigenvalue of the covariance Surrey TW20 0EX, UK matrix). A statistical F-test is used to assess the uniqueness of the ............................................................................................................................................................................. estimated splitting parameters and thereby provides an error The rifting of continents and evolution of ocean basins is a estimate10. fundamental component of plate tectonics, yet the process of The SKS splitting results obtained from the Ethiopian data are of continental break-up remains controversial. Plate driving forces exceptional quality and resolution (see Supplementary Information have been estimated to be as much as an order of magnitude for some examples and list of results). We have obtained a remark- smaller than those required to rupture thick continental litho- able 327 SKS splitting observations in a region focused on the sphere1,2. However, Buck1 has proposed that lithospheric heating Northern Ethiopian Rift. The anisotropy parameters are well by mantle upwelling and related magma production could constrained and we use a cutoff error criteria of ^0.6 s for dt and promote lithospheric rupture at much lower stresses. -
Constraints on the Tectonic Evolution of the Westernmost Mediterranean and Northwestern Africa from Shear Wave Splitting Analysis
Earth and Planetary Science Letters 375 (2013) 234–243 Contents lists available at ScienceDirect Earth and Planetary Science Letters journal homepage: www.elsevier.com/locate/epsl Constraints on the tectonic evolution of the westernmost Mediterranean and northwestern Africa from shear wave splitting analysis Meghan S. Miller a,n, Amir A. Allam a, Thorsten W. Becker a, Jeanette F. Di Leo b, James Wookey b a University of Southern California, Department of Earth Sciences, 3651 Trousdale Pkwy, Los Angeles, CA 90089, USA b University of Bristol, School of Earth Sciences, Wills Memorial Building, Queen's Road, Bristol BS8 1RJ, UK article info abstract Article history: The westernmost Mediterranean mantle and lithosphere have evolved into their current configuration Received 21 March 2013 due to complex interactions between the African and Eurasian plates. To help unravel the regional Received in revised form tectonics, we use new broadband seismic data across the Gibraltar arc and into southern Morocco to infer 17 May 2013 azimuthal seismic anisotropy and flow patterns for the upper mantle based on shear wave splitting Accepted 21 May 2013 analysis. A deep (4600 km) earthquake in April 2010 was recorded by the array and allowed us to Editor: P. Shearer Available online 10 July 2013 compare 31 direct S measurements with 235 teleseismic SK(K)S events from 3 years of deployment. The patterns of apparent fast polarization orientations and delay times suggest three major tectonic domains Keywords: when interpreted jointly with recent tomographic images of the subducted slab: (1) a subducted slab seismic anisotropy related toroidal flow domain centered upon the Alboran Sea and southern Spain, leading to complex subducted slab structure splits, (2), a region where the west African craton deflects mantle flow in the Anti-Atlas and High mantle-flow channeling craton–slab interaction Plateaux, and, (3), an intermediate domain across the central High Atlas. -
How Are Vertical Shear Wave Splitting Measurements Affected by Variations in the Orientation of Azimuthal Anisotropy with Depth?
Geophys. J. Int. (2000) 141, 374–390 How are vertical shear wave splitting measurements affected by variations in the orientation of azimuthal anisotropy with depth? Rebecca L. Saltzer, James B. Gaherty* and Thomas. H. Jordan Department of Earth, Atmospheric and Planetary Sciences, Massachusetts Institute of T echnology, Cambridge, MA 02139, USA. E-mail: [email protected] Accepted 1999 December 1. Received 1999 November 22; in original form 1999 May 20 SUMMARY Splitting measurements of teleseismic shear waves, such as SKS, have been used to estimate the amount and direction of upper mantle anisotropy worldwide. These measurements are usually made by approximating the anisotropic regions as a single, homogeneous layer and searching for an apparent fast direction (w˜) and an apparent splitting time (Dt˜) by minimizing the energy on the transverse component of the back- projected seismogram. In this paper, we examine the validity of this assumption. In particular, we use synthetic seismograms to explore how a vertically varying anisotropic medium affects shear wave splitting measurements. We find that weak heterogeneity causes observable effects, such as frequency dependence of the apparent splitting parameters. These variations can be used, in principle, to map out the vertical variations in anisotropy with depth through the use of Fre´chet kernels, which we derive using perturbation theory. In addition, we find that measurements made in typical frequency bands produce an apparent orientation direction that is consistently different from the average of the medium and weighted towards the orientation of the anisotropy in the upper portions of the model. This tendency of the measurements to mimic the anisotropy at the top part of the medium may explain why shear wave splitting measurements tend to be correlated with surface geology. -
A Review of Techniques for Measuring Shear-Wave Splitting Above Small
Physics of the Earth and Planetary Interiors 159 (2006) 1–14 Review A review of techniques for measuring shear-wave splitting above small earthquakes Stuart Crampin a,b,c,∗, Yuan Gao a,c a Shear-Wave Analysis Group, School of GeoSciences, University of Edinburgh, Grant Institute West Mains Road, Edinburgh EH9 3JW, Scotland, UK b Edinburgh Anisotropy Project, British Geological Survey, Murchison House, West Mains Road, Edinburgh EH9 3LA, Scotland, UK c Institute of Earthquake Science, China Earthquake Administration, 63 Fuxing Road, 100036 Beijing, China Received 9 November 2005; received in revised form 22 May 2006; accepted 15 June 2006 Abstract Seismic shear-wave splitting is difficult to measure accurately because of the complexity of the shear-wave signal. A variety of techniques have been developed for measuring time-delays and polarisations of shear-wave splitting above small earthquakes. These range from ‘display’ techniques, where measurements depend on visual examination of rotated seismograms and polarisation diagrams, through a range of increasingly automatic techniques, to what are almost fully automatic processes. All techniques have disadvantages. Visual techniques are subjective and, although arguably the most accurate, are tedious and time-consuming. More automated techniques work well on noise-free impulsive near-classic examples of shear-wave splitting, but on typical records either require visual checking or need to pass stringent selection criteria which may severely limit the data and bias the results. The accompanying paper presents a combination of visual and automatic techniques to provide a user-friendly semi-automatic measurement technique. Such techniques are important because the new understanding of fluid-rock deformation suggests that shear-wave splitting monitors the low-level deformation of fluid-saturated microcracks in hydrocarbon production processes, as well as the accumulation of stress before earthquakes, and other applications. -
Systematic Analysis of Shear-Wave Splitting in the Aftershock Zone of the 1999 Chi-Chi, Taiwan, Earthquake
Bulletin of the Seismological Society of America, Vol. 94, No. 6, pp. 2330–2347, December 2004 Systematic Analysis of Shear-Wave Splitting in the Aftershock Zone of the 1999 Chi-Chi, Taiwan, Earthquake: Shallow Crustal Anisotropy and Lack of Precursory Variations by Yunfeng Liu, Ta-Liang Teng, and Yehuda Ben-Zion Abstract We analyze shear-wave splitting (SWS) in a high-quality waveform data set recorded at surface and downhole (0.2 km) seismometers in a region around the 20 September 1999 Mw 7.6 Chi-Chi, Taiwan, earthquake sequence. The data set was generated by events in a 5-year period before, during, and after the mainshock. The purpose is to investigate the depth extent of the crustal anisotropy and its possible temporal evolution in relation to the occurrence of large earthquakes. Results from downhole records show a stable polarization direction of the fast shear wave that matches well the local Global Positioning System (GPS) velocity field. A slightly different polarization direction of the fast shear wave is obtained from surface data. This suggests a possible anisotropy change between the top 0.2 km structure and the deeper section of the crust. Measured time delays below the downhole station have an average value of 0.16 sec without systematic changes for sources from about 8 km to 20 km in depth. Estimates of time delays in the top 0.2 km of the crust based on shear waves reflected from the free surface give a constant 0.04 sec. A likely depth distribution inferred from these two types of measurements and an S-velocity model indicates that the crustal anisotropy in the region is dominated by the top 2 to 3 km. -
A Reappraisal of Shear Wave Splitting Parameters from Italian Active Volcanic Areas Through a Semiautomatic Algorithm Francesca Bianco, Lucia Zaccarelli
A reappraisal of shear wave splitting parameters from Italian active volcanic areas through a semiautomatic algorithm Francesca Bianco, Lucia Zaccarelli To cite this version: Francesca Bianco, Lucia Zaccarelli. A reappraisal of shear wave splitting parameters from Italian active volcanic areas through a semiautomatic algorithm. Journal of Seismology, Springer Verlag, 2008, 13 (2), pp.253-266. 10.1007/s10950-008-9125-z. hal-00478438 HAL Id: hal-00478438 https://hal.archives-ouvertes.fr/hal-00478438 Submitted on 30 Apr 2010 HAL is a multi-disciplinary open access L’archive ouverte pluridisciplinaire HAL, est archive for the deposit and dissemination of sci- destinée au dépôt et à la diffusion de documents entific research documents, whether they are pub- scientifiques de niveau recherche, publiés ou non, lished or not. The documents may come from émanant des établissements d’enseignement et de teaching and research institutions in France or recherche français ou étrangers, des laboratoires abroad, or from public or private research centers. publics ou privés. J Seismol (2009) 13:253–266 DOI 10.1007/s10950-008-9125-z ORIGINAL ARTICLE A reappraisal of shear wave splitting parameters from Italian active volcanic areas through a semiautomatic algorithm Francesca Bianco · Lucia Zaccarelli Received: 6 June 2007 / Accepted: 18 December 2007 / Published online: 11 September 2008 © Springer Science + Business Media B.V. 2008 Abstract Shear wave splitting parameters repre- of the fast polarization (ϕ) and percentage of sent a useful tool to detail the stress changes shear wave anisotropy (ξ). occurring in volcanic environments before im- pending eruptions. In the present paper, we dis- Keywords Shear wave splitting parameters · play the parameter estimates obtained through Temporal variations · Volcano seismology · implementation of a semiautomatic algorithm ap- Semiautomatic techniques plied to all useful datasets of the following Italian active volcanic areas: Mt. -
Automation of Shear-Wave Splitting Parameter Determination of Local Earthquakes at Yellowstone : Application As Indicator of Crustal Stress and Temporal Variation
Michigan Technological University Digital Commons @ Michigan Tech Dissertations, Master's Theses and Master's Dissertations, Master's Theses and Master's Reports - Open Reports 2011 Automation of shear-wave splitting parameter determination of local earthquakes at Yellowstone : application as indicator of crustal stress and temporal variation Nicole D. McMahon Michigan Technological University Follow this and additional works at: https://digitalcommons.mtu.edu/etds Part of the Geophysics and Seismology Commons Copyright 2011 Nicole D. McMahon Recommended Citation McMahon, Nicole D., "Automation of shear-wave splitting parameter determination of local earthquakes at Yellowstone : application as indicator of crustal stress and temporal variation ", Master's Thesis, Michigan Technological University, 2011. https://doi.org/10.37099/mtu.dc.etds/333 Follow this and additional works at: https://digitalcommons.mtu.edu/etds Part of the Geophysics and Seismology Commons AUTOMATION OF SHEAR-WAVE SPLITTING PARAMETER DETERMINATION OF LOCAL EARTHQUAKES AT YELLOWSTONE: APPLICATION AS INDICATOR OF CRUSTAL STRESS AND TEMPORAL VARIATION By Nicole D. McMahon A THESIS Submitted in partial fulfillment of the requirements for the degree of MASTER OF SCIENCE (Geophysics) MICHIGAN TECHNOLOGICAL UNIVERSITY 2011 © 2011 Nicole D. McMahon This thesis, “Automation of shear-wave splitting parameter determination of local earthquakes at Yellowstone: Application as indicator of crustal stress and temporal variation,” is hereby approved in partial fulfillment of the requirements for the Degree of MASTER OF SCIENCE IN GEOPHYSICS. Department of Geological and Mining Engineering and Sciences Signatures: Thesis Advisor _____________________________________________ Dr. Gregory P. Waite Committee Member _____________________________________________ Dr. Simon A. Carn Committee Member _____________________________________________ Dr. Petra Huentemeyer Department Chair _____________________________________________ Dr. Wayne D.