National Science and Implementation Plan 2015-25
Total Page:16
File Type:pdf, Size:1020Kb
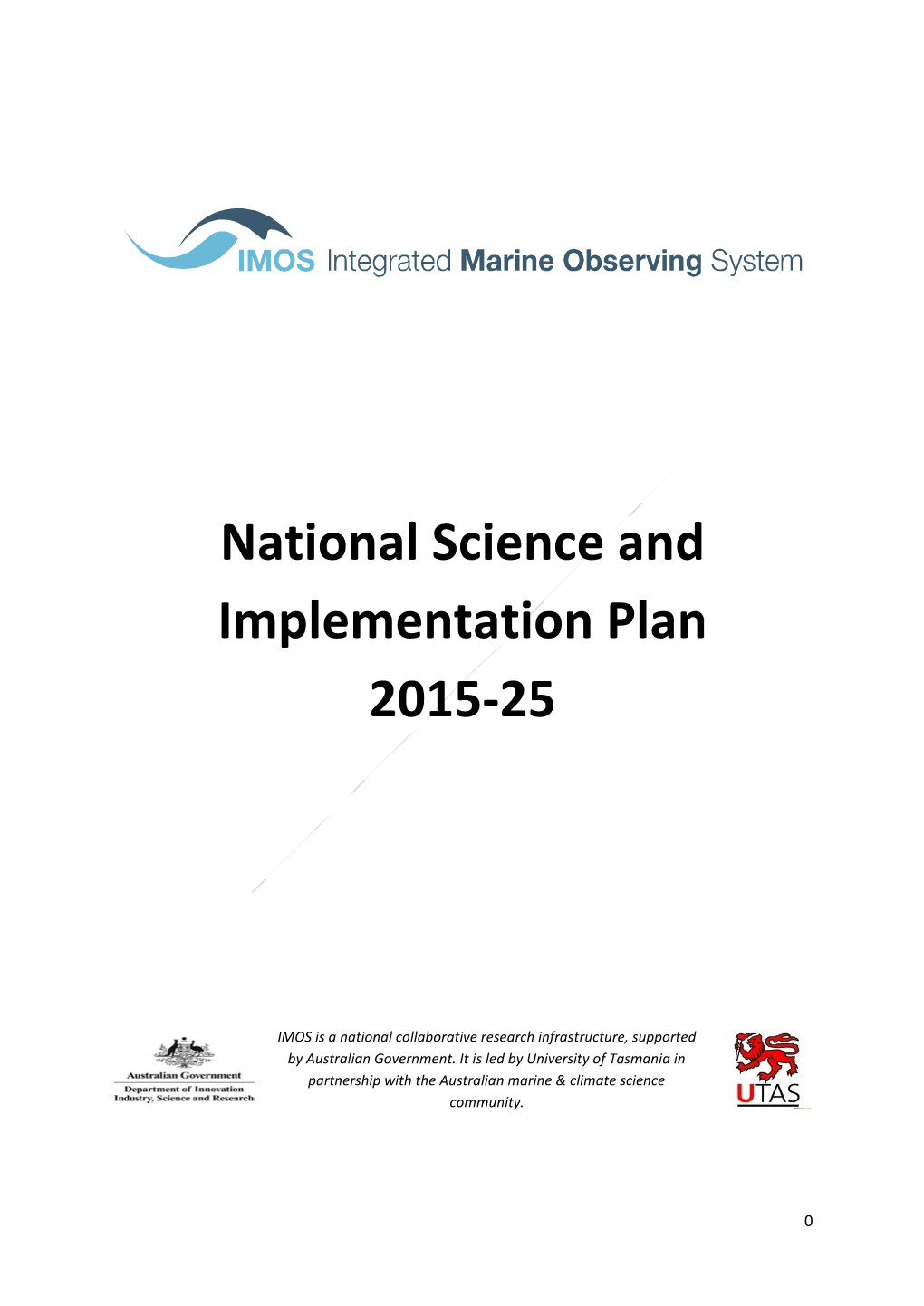
Load more
Recommended publications
-
Variability in Ocean Currents Around Australia
State and Trends of Australia’s Oceans Report 1.4 Variability in ocean currents around Australia Charitha B. Pattiaratchi1,2 and Prescilla Siji1,2 1 Oceans Graduate School, The University of Western Australia, Perth, WA, Australia 2 UWA Oceans Institute, The University of Western Australia, Perth, WA, Australia Summary Ocean currents also have a strong influence on marine ecosystems, through the transport of heat, nutrients, phytoplankton, zooplankton and larvae of most marine animals. We used geostrophic currents derived satellite altimetry measurements between 1993 and 2019 to examine the Kinetic Energy (KE, measure of current intensity) and Eddy Kinetic Energy (EKE, variability of the currents relative to a mean) around Australia. The East Australian (EAC) and Leeuwin (LC) current systems along the east and west coasts demonstrated strong seasonal and inter-annual variability linked to El Niño and La Niña events. The variability in the LC system was larger than for the EAC. All major boundary currents around Australia were enhanced during the 2011 La Niña event. Key Data Streams State and Trends of Australia’s Ocean Report www.imosoceanreport.org.au Satellite Remote Time-Series published Sensing 10 January 2020 doi: 10.26198/5e16a2ae49e76 1.4 I Cuirrent variability during winter (Wijeratne et al., 2018), with decadal ENSO Rationale variations affecting the EAC transport variability (Holbrook, Ocean currents play a key role in determining the distribution Goodwin, McGregor, Molina, & Power, 2011). Variability of heat across the planet, not only regulating and stabilising associated with ENSO along east coast of Australia appears climate, but also contributing to climate variability. Ocean to be weaker than along the west coast. -
Tasman Leakage: a New Route in the Global Ocean Conveyor Belt
Tasman leakage: a new route in the global ocean conveyor belt Sabrina Speich, Bruno Blanke, Pedro de Vries, Sybren Drijfhout, Kristofer Döös, Alexandre Ganachaud, Robert Marsh To cite this version: Sabrina Speich, Bruno Blanke, Pedro de Vries, Sybren Drijfhout, Kristofer Döös, et al.. Tasman leakage: a new route in the global ocean conveyor belt. Geophysical Research Letters, American Geophysical Union, 2002, 29 (10), pp.55-1-55-4. 10.1029/2001GL014586. hal-00172866 HAL Id: hal-00172866 https://hal.archives-ouvertes.fr/hal-00172866 Submitted on 27 Jan 2021 HAL is a multi-disciplinary open access L’archive ouverte pluridisciplinaire HAL, est archive for the deposit and dissemination of sci- destinée au dépôt et à la diffusion de documents entific research documents, whether they are pub- scientifiques de niveau recherche, publiés ou non, lished or not. The documents may come from émanant des établissements d’enseignement et de teaching and research institutions in France or recherche français ou étrangers, des laboratoires abroad, or from public or private research centers. publics ou privés. GEOPHYSICAL RESEARCH LETTERS, VOL. 29, NO. 10, 1416, 10.1029/2001GL014586, 2002 Tasman leakage: A new route in the global ocean conveyor belt Sabrina Speich and Bruno Blanke Laboratoire de Physique des Oce´ans, Brest, France Pedro de Vries and Sybren Drijfhout Royal Netherlands Meteorological Institute, De Bilt, The Netherlands Kristofer Do¨o¨s Meteorologiska Institutionen, Stocholms Universitet, Stockholm, Sweden Alexandre Ganachaud Institut de Recherche pour le De´veloppement, Laboratoire d’E´ tudes en Ge´ophysique et Oce´anographie Spatiale, Toulouse, France Robert Marsh James Rennell Division for Ocean Circulation and Climate, Southampton Oceanography Centre, European Way, United Kingdom Received 18 December 2001; accepted 15 March 2002; published 24 May 2002. -
Q-IMOS: the Queensland Node of the Integrated Marine Observing System
Q‐IMOS: The Queensland Node of the Integrated Marine Observing System Peter Doherty (AIMS) and Russ Babcock (CSIRO) Q-IMOS fixed infrastructure Slope moorings Shelf moorings SEC National Reference Stations –real time HF radar –real time Satellite dish (SST, OC) Island Research Stations – wireless sensor networks – real time Reef towers –real time Acoustic receivers EAC moorings Lucinda Jetty Coastal Observatory Q-IMOS mobile infrastructure •SOOP (& CPR) •Gliders •AUV IMOS Research Themes Global Energy Budget Pacific Ocean Global Hydrological Cycle Indian Ocean Global Circulation Southern Ocean Global Carbon Cycle 1. Multi‐decadal ocean change Fluxes Interannual Drivers intraseasonal Dynamics 3. Major 2. Climate boundary 5. Ecosystem responses productivity, abundance, distribution variability currents and weather extremes and inter‐basin flows nutrients microbes plankton nekton benthic East Australian Current ENSO, IOD, SAM, MJO apex predators pelagic (+Tasman Outflow, Flinders Cyclones, ECL’s Current, Hiri Current) Leeuwin Current Indonesian Throughflow Antarctic Circumpolar 4. Continental Current shelf processes eddy encroachment upwelling, downwelling cross shelf exchange coastal currents wave climate A. Ganachaud1, 2, Bowen M.3, Brassington G.4, Cai W.5, Cravatte S.2,1, Davis R. 6, Gourdeau L.1, Hasegawa T. 7, Hill K.8,9, Holbrook N.10, Kessler W. 11, Maes C.1 , Melet A.12,13, Qiu B.14, Ridgway, K.15, Roemmich D. 6, Schiller A.15, Send U. 6, Sloyan B.15, Sprintall J.6, Steinberg C.16, Sutton P. 17, Verron J.12, Widlansky M.14,18, Wiles P. 19 (2013) CLIVAR Newsletter SPICE Community CLIVAR Exchanges No. 58, Vol. 17, No.1, February 2012 Waypoints for Sea Glider missions 22 Oct 2012 (99 days at June‐Oct 2010 (149 days) last surfacing 28 Jan 2013) IMOS Research Themes Global Energy Budget Pacific Ocean Global Hydrological Cycle Indian Ocean Global Circulation Southern Ocean Global Carbon Cycle 1. -
Wintertime Circulation Off Southeast Australia: Strong Forcing by the East Australian Current John F
JOURNAL OF GEOPHYSICAL RESEARCH, VOL. 110, C12012, doi:10.1029/2004JC002855, 2005 Wintertime circulation off southeast Australia: Strong forcing by the East Australian Current John F. Middleton School of Mathematics, University of New South Wales, Sydney, New South Wales, Australia Mauro Cirano Centro de Pesquisa em Geofisica e Geologia, Universidade Federal da Bahia, Salvador, Brazil Received 21 December 2004; revised 18 April 2005; accepted 22 September 2005; published 13 December 2005. [1] Numerical results and observations are used to examine the sub–weather band wintertime circulation off the eastern shelf slope region between Tasmania and Cape Howe. The numerical model is forced using wintertime-averaged atmospheric fluxes of momentum, heat, and freshwater and using transports along open boundaries that are obtained from a global ocean model. The boundary to the north of Cape Howe has a prescribed transport of 17 Sv, which from available observations represents strong forcing by the East Australian Current (EAC). Nonetheless, the model results are in qualitative (and quantitative) agreement with several observational studies. In accord with observations, water within Bass Strait is found to be driven to the east and north with speeds of up to 30 cm sÀ1 off the southeast Victorian coast. The water is colder and denser than that found farther offshore, which is associated with the southward flowing (warm) EAC. In agreement with observations the density difference between the two water masses is about 0.6 kg mÀ3 and leads to a cascade of dense Bass Strait water to depths of 300 m and as a plume that extends 5–10 km in the cross-shelf direction. -
Analysis of Hydrographic and Stable Isotope Data to Determine Water Masses, Circulation, and Mixing in the Eastern Great Australian Bight Laura E
JOURNAL OF GEOPHYSICAL RESEARCH, VOL. 114, C10016, doi:10.1029/2009JC005407, 2009 Analysis of hydrographic and stable isotope data to determine water masses, circulation, and mixing in the eastern Great Australian Bight Laura E. Richardson,1 T. Kurt Kyser,2 Noel P. James,2 and Yvonne Bone3 Received 26 March 2009; revised 29 July 2009; accepted 6 August 2009; published 17 October 2009. [1] Hydrographic and stable isotope data from waters in the eastern Great Australian Bight (GAB) sampled during March–April 1998 indicate that both mixing and evaporative processes are important on the shelf. Five water masses are defined on the basis of their temperature, salinity, d2H and d18O values. Two of these are end-members, the Flinders Current (FC) and the Great Australian Bight Plume (GABP), whereas the other three are a result of mixing between these two end-members. Water mass distribution reflects an anticyclonic gyre in the eastern GAB. Cool and fresh water present at depth along the Eyre Peninsula is sourced from upwelling of Flinders Current water directly from the shelf break. This water is progressively heated, evaporated, and mixed with warmer and more saline shelf waters as it flows around the gyre. High temperatures, salinities, and d2H values in surface waters in the central GAB suggest that the Great Australian Bight Plume has a greater spatial extent than previously recorded, also occurring along the shelf edge between 130°E and 133°E. A high temperature, high salinity, low d2H water mass that is isotopically similar to the Flinders Current occurs in the west of the study area, indicating intrusion of Flinders Current water into the central GAB. -
The Southwest Pacific Ocean Circulation and Climate Experiment
VolumeVolume No.13 9 No.4 No.33 September OctoberSeptember 20082004 2004 CLIVAR Exchanges The Southwest PacIfic Ocean Circulation and Climate Experiment (SPICE): a new CLIVAR programme Ganachaud, A.1, W. Kessler2, G. Brassington3, C. R. Mechoso4, S. Wijffels5, K. Ridgway5, W. Cai6, N. Holbrook7,8, P. Sutton9, M. Bowen9, B. Qiu10, A. Timmermann10, D. Roemmich11, J. Sprintall11, D. Neelin4, B. Lintner4, H. Diamond12, S. Cravatte13, L. Gourdeau1, P. Eastwood14, T. Aung15 1Laboratoire d’Etudes en Géophysique et Océanographie Spatiales (LEGOS)/Institut de Recherche pour le Développement (IRD), Nouméa, New Caledoni; 2National Oceanic and Atmospheric Administration (NOAA)/Pacific Marine Environmental; Laboratory (PMEL), Seattle, WA, USA; 3Centre for Australian Weather and Climate Research, A partnership between the Australian; Bureau of Meteorology and CSIRO, Melbourne, Australia, 3001; 4Department of Atmospheric Sciences, University of California, Los Angeles, CA, USA; 5CSIRO Marine and Atmospheric Research, Hobart, Australia; 6CSIRO Marine and Atmospheric Research, Aspendale, Australia; 7School of Geography and Environmental Studies, University of Tasmania, Hobart, Tasmania, Australia; 8Centre for Marine Science, University of Tasmania, Hobart; Tasmania, Australia; 9NIWA, Wellington, New Zealand; 10University of Hawaii, Honolulu, HI, USA; 11Scripps Institution of Oceanography, San Diego, CA, USA; 12NOAA/National Climatic Data Center, Silver Spring, Maryland, USA; 13Laboratoire d’Etudes en Géophysique et Océanographie Spatiales (LEGOS)/Institut -
Baroclinic Transport Variability of the Antarctic Circumpolar Current South of Australia (WOCE Repeat Section SR3) Stephenr
JOURNAL OF GEOPHYSICAL RESEARCH, VOL. 106, NO. C2, PAGES 2815-2832, FEBRUARY 15, 2001 Baroclinic transport variability of the Antarctic Circumpolar Current south of Australia (WOCE repeat section SR3) StephenR. Rintoul and SergueiSokolov CSIRO Marine Research and Antarctic Cooperative Research Centre Hobart, Tasmania, Australia Abstract. Baroclinic transport variability of the Antarctic Circumpolar Current (ACC) near 140øEis estimatedfrom six occupationsof a repeat sectionoccupied as part of the World OceanCirculation Experiment (WOCE sectionSR3). The meantop-to-bottom volume transport is 147+10 Sv (mean+l standarddeviation), relative to a deep referencelevel consistentwith water mass properties and float trajectories. The location and transport of the main fronts of the ACC are relatively steady: the Subantarctic Front carries 105+7 Sv at a mean latitude between 51o and 52øS;the northern branch of the Polar Front carries 5+5 Sv to the east between 53ø and 54øS;the southern Polar Front carries 24+3 Sv eastward at 59øS; and two cores of the southern ACC front at 62ø and 64øS carry 18+3 and ll+3 Sv, respectively. The variability in net property transports is largely due to variability of currents north of the ACC, in particular, an outflow of 8+13 Sv of water from the Tasman Sea and a deep anticyclonicrecirculation carrying 22+8 Sv in the Subantarctic Zone. Variability of net baroclinic volume transport is similar in magnitude to that measuredat Drake Passage.In density layers, transport variability is small in deeplayers, but significant(range of 4 to 16 Sv) in the SubantarcticMode Water. Variability of eastwardheat transportacross SR3 is significant(range of 139øCSv, or 0.57x 10•5 W, relativeto 0øC)and large relative to meridionalheat flux in the Southern Hemispheresubtropical gyres. -
Presentation
Ocean circulation and the mass and heat budgets of large ocean regions D. Roemmich and J. Gilson Scripps Institution of Oceanography, UCSD First XBT Science Workshop Melbourne July 7‐8, 2011 Outline Upstream Kuroshio near Taiwan • PtPart I: The Hig h RltiResolution XBT (HRX) NtNetwork samples the world’s boundary currents ‐ the subtropical WBCs and EBCs, the low latitude WBCs, and the ACC. – Global in scope (i.e. all 5 subtropical WBCs) – Enhanced BC sampling is highest priority, OO’09. – Argo provides complementary absolute and/or deep relative reference level velocities. – The HRX Network integrates the BCs and interior. • PtPart II: Clos ing the mass and htheat bdbudge ts of large ocean regions –the North Pacific example. – Mean budgets close, with a shallow overturning circulation of 13 Sv and 0750.75 pW of heat transport into the region north of PX37/10/44. – Time‐varying budgets remain a challenge, but HRX Line PX37/10/44 significant interannual variability is seen during the well‐measured Argo era: ~3 Sv in volume transport and ~.2 pW. HRX: Observing the world’s boundary current systems : Boundary currents sampled by the HRX Network HRX transects are sampling: • KhiKuroshio (3 HRX tk)tracks) • Gulf Stream (3 HRX tracks) • Agulhas • Brazil Current AOML status map • East Australian Current (2 HRX tracks) • East Auckland Current and Tasman Outflow • Eastern boundary currents (California Current, Alaska Current, Leeuwin Current, …) • Low latitude WBCs: Solomon Sea, Indonesian Throughflow • Antarctic Circumpolar Current (3 HRX tracks) • … “Upstream” Kuroshio near Taiwan, PX44 “Downstream” Kuroshio near Yokohama, PX05 w/Argo ref. velocity; 0-800m Kuroshio 21 Sv (120oE w/Argo ref. -
Southwest Pacific Ocean Circulation and Climate Experiment: SPICE A
Southwest PacIfic Ocean Circulation and Climate Experiment: SPICE A. Ganachaud JISAO/PMEL/NOAA LEGOS / IRD Nouméa-Toulouse Laboratoire d’Etudes Géophysiques et d’Océanographie Spatiale W. Kessler (PMEL/NOAA) G. Brassington(BOM) S. Wijffels, K. Ridgway, W. Cai (CSIRO) N. Holbrook (MU) P. Sutton, M. Bowen (NIWA) B. Qiu, A. Timmermann (UH) D. Roemmich, J. Sprintall (SIO), H. Diamond (NOAA) S. Cravatte, L. Gourdeau (LEGOS) P. Eastwood (SOPAC/Fiji) Black (1851) T. Aung (USP/Fiji) Outline A. Thermocline waters B. SPCZ SOLOMON IS. C. Impacts PNG Solomon D. Context and strategy Sea VANUATU Coral Fiji Sea N. CALEDONIA AUSTRALIA Tasman Sea NEW ZEALAND Rotschi, Legand, Hamon Interests in the Coral and Tasman Sea circulation R/V ORSOM III, Cronulla, August 1958 1956 Conference on the Coral and Tasman Sea interisland Oceanography flows Cairns, August 2005 Workshop on the Southwest Pacific Ocean Circulation and its relation with Climate Decadal influences: Thermocline water connection between the subduction zone of the South East Pacific and the equator Lines show geostrophic streamlines on the isopycnal (courtesy B. Kessler) A-The South Pacific "Thermocline" waters South Equatorial Current Salinity maximum Z=100:300m Temperature/salinity diagram (Argo floats, 2007) Thermocline water currents: 0-300m ~25 Sv Thermocline water currents 0-300m 70 % EUC waters ~15 Sv + ~25 Sv Thermocline water currents 0-300m ~15 Sv + ~25 Sv ~10 Sv Thermocline water currents 0-300m 3-Solomon Sea 1-Inflows 2-Tasman Sea Thermocline waters: 1-inflow to the Coral Sea 1. Formation of 3 "jets" 2. Bifurcation (northward bias in GCM/CGCMs) 3. Outflows and budget Bifurcation: Qu and Lindstrom 2002 Kessler and Gourdeau 2006, 2007 Thermocline waters: 2-EAC and EAUC Ridgway and Dunn, (2003); Cai et al. -
The East Australian Current (EAC) Is a Complex and Highly Energetic Western Boundary System in the South-Western Pacific Off Eastern Australia
See discussions, stats, and author profiles for this publication at: https://www.researchgate.net/publication/228492387 The East Australian Current Article · January 2009 CITATIONS READS 44 4,117 2 authors, including: Ken Ridgway CSIRO Oceans and Atmosphere, Hobart, Australia 68 PUBLICATIONS 5,175 CITATIONS SEE PROFILE Some of the authors of this publication are also working on these related projects: Sensor networks View project Coral Sea review View project All content following this page was uploaded by Ken Ridgway on 31 May 2014. The user has requested enhancement of the downloaded file. The East Australian Current Ken Ridgway1, Katy Hill2 1CSIRO Marine and Atmospheric Research, PO Box 1538, Hobart, TAS 7001, Australia 2Integrated Marine Observing System, University of Tasmania, Private Bag 110, Hobart, TAS 7001, Australia. Ridgway, K. and Hill, K. (2009) The East Australian Current. In A Marine Climate Change Impacts and Adaptation Report Card for Australia 2009 (Eds. E.S. Poloczanska, A.J. Hobday and A.J. Richardson), NCCARF Publication 05/09, ISBN 978-1-921609-03-9. Lead author email: [email protected] Summary: The East Australian Current (EAC) is a complex and highly energetic western boundary system in the south-western Pacific off eastern Australia. The EAC provides both the western boundary of the South Pacific Gyre and the linking element between the Pacific and Indian Ocean gyres. The EAC is weaker than other western boundary currents and is dominated by a series of mesoscale eddies that produce highly variable patterns of current strength and direction. Seasonal, interannual and strong decadal changes are observed in the current, which tend to mask the underlying long-term trends related to greenhouse gas forcing. -
Southern Australian Integrated Marine Observing System (SAIMOS) Node Science and Implementation Plan 2015-25
Southern Australian Integrated Marine Observing System (SAIMOS) Node Science and Implementation Plan 2015-25 IMOS is a national collaborative research infrastructure, supported by Australian Government. It is led by University of Tasmania in partnership with the Australian marine & climate science community. 1 IMOS Node Southern Australian Integrated Marine Observing System Lead Institution South Australian Research and Development Institute (SARDI) Name: Simon D. Goldsworthy Affiliation: SARDI Node Leader Address: 2 Hamra Avenue, West Beach, SA 5024 Phone: 08 8207 5325 Email: [email protected] Name: Sophie C. Leterme Affiliation: Flinders University Deputy Node Leader Address: Sturt Road, Bedford Park, SA 5042 Phone: 08 8201 3774 Email: [email protected] These include: SA Dept. Environment, Water and Natural Resources (DEWNR); Primary Industries and Regions SA; DEPI Vic; ANU, Dept. of Collaborating Institutions the Environment; Curtin University; Deakin University; Macquarie University; Sydney Institute of Marine Science (SIMS); the Institute for Marine and Antarctic Studies (IMAS). Lead authors: P. van Ruth, M. Doubell, S. Goldsworthy, C. Huveneers, J. Middleton, P. Rogers Contributing authors: P. Fairweather, P Gill, G. Hays, P. Hesp, C. James, N. James, S. Leterme, A Levings, M. Loo, J. Luick, G. Miot Da Silva, M. Morrice, L. Seuront, S. Sorokin, T. Ward Edited by: S.D. Goldsworthy and S.C. Leterme Date: 26/05/2014 2 Table of Contents Table of Contents ................................................................................................................................... -
(NSW-IMOS) Node Science and Implementation Plan 2015-25
New South Wales Integrated Marine Observing System (NSW-IMOS) Node Science and Implementation Plan 2015-25 IMOS is a national collaborative research infrastructure, supported by Australian Government. It is led by University of Tasmania in partnership with the Australian marine & climate science community. 1 IMOS Node New South Wales Integrated Marine Observing System Node Lead Institution Sydney Institute of Marine Science (SIMS) Name: Assoc Prof Martina Doblin Affiliation: University of Technology Sydney/SIMS Node Leader(s) Address: PO Box 123 Broadway, Ultimo, NSW 2007 Phone: 02 9514 8307 Email: [email protected] Name: Dr Tim Ingleton Affiliation: New South Wales Office of Environment & Heritage/SIMS/Research Associate IMAS University of Tasmania Address: 59-61 Goulburn Street Sydney South 1232 Phone: 02 9995 5517 Deputy Node Leader(s) Email: [email protected] Name: Dr Robin Robertson Affiliation: University of New South Wales Canberra/SIMS Address: School of PEMS; PO Box 7961 BC; Canberra, ACT; 2610 Phone: 02 6268 8289 Email: [email protected] University of New South Wales; Macquarie University; University of Sydney; University of Technology Sydney; NSW Department of Collaborating Institutions Environment, Climate Change & Water (DECCW); NSW Department of Service Technology and Administration; NSW Department of Industry and Investment; Sydney Water Corporation. Lead authors: Robin Robertson, Tim Ingleton, Martina Doblin, Iain Suthers, Moninya Roughan Contributing authors: Matt Taylor, Alan Jordan, Robert Harcourt, Jason Everett, Stefan Williams, Acacia Pepler, Agata Imielska The current authors would like to acknowledge the tremendous effort put in by the team who generated the previous NSW node plan in 2010.