CHRISTIAN HEISS I. Synthesis and Evaluation of Mechanism Based Inhibitors of Kynureninase
Total Page:16
File Type:pdf, Size:1020Kb
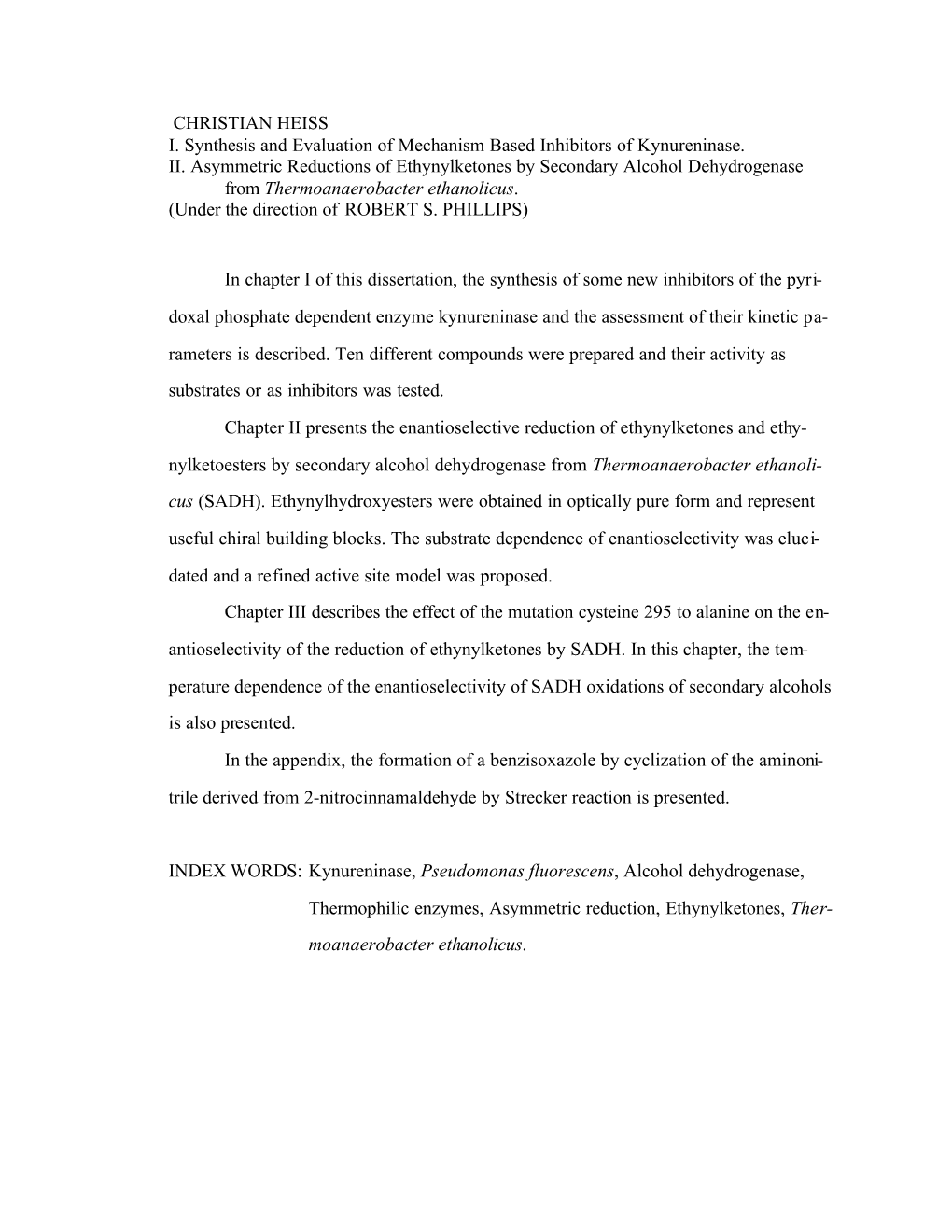
Load more
Recommended publications
-
The Excitotoxin Quinolinic Acid Induces Tau Phosphorylation in Human Neurons
The Excitotoxin Quinolinic Acid Induces Tau Phosphorylation in Human Neurons Abdur Rahman1,4., Kaka Ting2, Karen M. Cullen3, Nady Braidy4, Bruce J. Brew2,5, Gilles J. Guillemin2,4.* 1 Department of Family Sciences, College for Women, Kuwait University, Shuwaikh, Kuwait, 2 St Vincent’s Hospital, Centre for Applied Medical Research, Department of Neuroimmunology, Darlinghurst, New South Wales, Australia, 3 Disciplines of Anatomy and Histology, School of Medical Science, The University of Sydney, New South Wales, Australia, 4 Department of Pharmacology, University of New South Wales, School of Medical Science, Sydney, New South Wales, Australia, 5 Department of Neurology, St Vincent’s Hospital, Darlinghurst, New South Wales, Australia Abstract Some of the tryptophan catabolites produced through the kynurenine pathway (KP), and more particularly the excitotoxin quinolinic acid (QA), are likely to play a role in the pathogenesis of Alzheimer’s disease (AD). We have previously shown that the KP is over activated in AD brain and that QA accumulates in amyloid plaques and within dystrophic neurons. We hypothesized that QA in pathophysiological concentrations affects tau phosphorylation. Using immunohistochemistry, we found that QA is co-localized with hyperphosphorylated tau (HPT) within cortical neurons in AD brain. We then investigated in vitro the effects of QA at various pathophysiological concentrations on tau phosphorylation in primary cultures of human neurons. Using western blot, we found that QA treatment increased the phosphorylation of tau at serine 199/202, threonine 231 and serine 396/404 in a dose dependent manner. Increased accumulation of phosphorylated tau was also confirmed by immunocytochemistry. This increase in tau phosphorylation was paralleled by a substantial decrease in the total protein phosphatase activity. -
Download Product Insert (PDF)
Product Information Quinolinic Acid Item No. 14941 CAS Registry No.: 89-00-9 Formal Name: 2,3-pyridinedicarboxylic acid Synonyms: NSC 13127, NSC 18836, NSC 403247 O N MF: C7H5NO4 FW: HO 167.1 HO Purity: ≥98% Stability: ≥2 years at -20°C Supplied as: A crystalline solid O λ UV/Vis.: max: 216, 264 nm Laboratory Procedures For long term storage, we suggest that quinolinic acid be stored as supplied at -20°. It should be stable for at least two years. Quinolinic acid is supplied as a crystalline solid. A stock solution may be made by dissolving the quinolinic acid in the solvent of choice. Quinolinic acid is soluble in organic solvents such as DMSO and dimethyl formamide, which should be purged with an inert gas. The solubility of quinolinic acid in these solvents is approximately 16 mg/ml. Further dilutions of the stock solution into aqueous buffers or isotonic saline should be made prior to performing biological experiments. Ensure that the residual amount of organic solvent is insignificant, since organic solvents may have physiological effects at low concentrations. Organic solvent-free aqueous solutions of quinolinic acid can be prepared by directly dissolving the crystalline solid in aqueous buffers. The solubility of quinolinic acid in PBS, pH 7.2, is approximately 0.5 mg/ml. We do not recommend storing the aqueous solution for more than one day. Quinolinic acid is an endogenous agonist at NMDA receptors that is generated through the metabolism of tryptophan in the kynurenine pathway.1 By overactivating NMDA receptors, quinolinic acid produces neurotoxicity, which has been implicated in certain neurodegenerative disorders.2 Quinolinic acid can also generate reactive oxygen species, has immunomodulatory actions, and promotes the formation of hyperphosphorylated tau proteins.3-5 References 1. -
Expression and Regulation of the Rate-Limiting Enzymes of the Kynurenine Pathway in the Mouse Brain by Alexandra Kelly Brooks Di
EXPRESSION AND REGULATION OF THE RATE-LIMITING ENZYMES OF THE KYNURENINE PATHWAY IN THE MOUSE BRAIN BY ALEXANDRA KELLY BROOKS DISSERTATION Submitted in partial fulfillment of the requirements for the degree of Doctor of Philosophy in Neuroscience in the Graduate College of the University of Illinois at Urbana-Champaign, 2017 Urbana, Illinois Doctoral Committee: Research Assistant Professor Robert H. McCusker, Chair Assistant Professor Andrew J. Steelman Assistant Professor Monica Uddin Professor Rodney W. Johnson P a g e | ii ABSTRACT During the mid-1900’s, early anti-depressants were developed to increase levels of catecholamines within the brain, leading to the theory that depression symptomology was a result of an imbalance of neurotransmitters within the brain. To date, the catecholamine theory of depression is still held to be the prevailing theory as selective serotonin reuptake inhibitors being the most commonly prescribed anti-depressants. However, the incidence of depression is still rising with a vast amount of patients failing to respond to current treatments, and with this knowledge, additional theories of the neurobiology of depression have arisen over the past 30 years. A leading theory is Kynurenine Pathway activation in relation to inflammation- or stress- induced depression-like behaviors. There has been a tremendous amount of data connecting activated immune system (or hypothalamic-pituitary-adrenal axis), increased kynurenine production and depression symptomology. Thus, understanding the factors that activate this -
Linking Phencyclidine Intoxication to the Tryptophan-Kynurenine Pathway Therapeutic Implications for Schizophrenia
Neurochemistry International 125 (2019) 1–6 Contents lists available at ScienceDirect Neurochemistry International journal homepage: www.elsevier.com/locate/neuint Linking phencyclidine intoxication to the tryptophan-kynurenine pathway: Therapeutic implications for schizophrenia T ∗ Hidetsugu Fujigakia, ,1, Akihiro Mourib,c,1, Yasuko Yamamotoa, Toshitaka Nabeshimac,d, Kuniaki Saitoa,c,d,e a Department of Disease Control and Prevention, Fujita Health University Graduate School of Health Sciences, 1-98 Dengakugakubo, Kutsukake-cho, Toyoake, Aichi 470- 1192, Japan b Department of Regulatory Science, Fujita Health University Graduate School of Health Sciences, 1-98 Dengakugakubo, Kutsukake-cho, Toyoake, Aichi 470-1192, Japan c Japanese Drug Organization of Appropriate Use and Research, 3-1509 Omoteyama, Tenpaku-ku, Nagoya, Aichi 468-0069, Japan d Advanced Diagnostic System Research Laboratory, Fujita Health University Graduate School of Health Sciences, 1-98 Dengakugakubo, Kutsukake-cho, Toyoake, Aichi 470-1192, Japan e Human Health Sciences, Graduate School of Medicine and Faculty of Medicine, Kyoto University, 54 Shogoinkawahara-cho, Sakyo-ku, Kyoto 606-8507, Japan ARTICLE INFO ABSTRACT Keywords: Phencyclidine (PCP) is a dissociative anesthetic that induces psychotic symptoms and neurocognitive deficits in Phencyclidine rodents similar to those observed in schizophrenia patients. PCP administration in healthy human subjects in- Kynurenic acid duces schizophrenia-like symptoms such as positive and negative symptoms, and a range of cognitive deficits. It Quinolinic acid has been reported that PCP, ketamine, and related drugs such as N-methyl-D-aspartate-type (NMDA) glutamate Kynurenine pathway receptor antagonists, induce behavioral effects by blocking neurotransmission at NMDA receptors. Further, Schizophrenia NMDA receptor antagonists reproduce specific aspects of the symptoms of schizophrenia. -
Tryptophan and Kynurenine Enhances the Stemness and Osteogenic Differentiation of Bone Marrow-Derived Mesenchymal Stromal Cells in Vitro and in Vivo
materials Article Tryptophan and Kynurenine Enhances the Stemness and Osteogenic Differentiation of Bone Marrow-Derived Mesenchymal Stromal Cells In Vitro and In Vivo Hai Thanh Pham 1,2 , Mitsuaki Ono 3,*, Emilio Satoshi Hara 4,* , Ha Thi Thu Nguyen 1,2,3, Anh Tuan Dang 1,2,3, Hang Thuy Do 1,2,3, Taishi Komori 1, Ikue Tosa 1, Yuri Hazehara-Kunitomo 1,3, Yuya Yoshioka 1, Yasutaka Oida 1, Kentaro Akiyama 1 and Takuo Kuboki 1 1 Department of Oral Rehabilitation and Regenerative Medicine, Okayama University Graduate School of Medicine, Dentistry and Pharmaceutical Sciences, Okayama 700-8558, Japan; [email protected] (H.T.P.); [email protected] (H.T.T.N.); [email protected] (A.T.D.); [email protected] (H.T.D.); [email protected] (T.K.); [email protected] (I.T.); [email protected] (Y.H.-K.); [email protected] (Y.Y.); [email protected] (Y.O.); [email protected] (K.A.); [email protected] (T.K.) 2 Faculty of Dentistry, Hai Phong University of Medicine and Pharmacy, Haiphong 04211, Vietnam 3 Department of Molecular Biology and Biochemistry, Okayama University Graduate School of Medicine, Dentistry and Pharmaceutical Sciences, Okayama 700-8558, Japan 4 Department of Biomaterials, Okayama University Graduate School of Medicine, Dentistry and Pharmaceutical Sciences, Okayama 700-8558, Japan * Correspondence: [email protected] (M.O.); [email protected] (E.S.H.); Tel.: +81-86-235-7127 (M.O.); +81-86-235-6667 (E.S.H.); Fax: +81-86-222-7768 (M.O.); +81-86-235-6669 (E.S.H.) Abstract: Aging tissues present a progressive decline in homeostasis and regenerative capacities, Citation: Pham, H.T.; Ono, M.; Hara, which has been associated with degenerative changes in tissue-specific stem cells and stem cell E.S.; Nguyen, H.T.T.; Dang, A.T.; Do, niches. -
Systemic Approaches to Modifying Quinolinic Acid Striatal Lesions in Rats
The Journal of Neuroscience, October 1988, B(10): 3901-3908 Systemic Approaches to Modifying Quinolinic Acid Striatal Lesions in Rats M. Flint Beal, Neil W. Kowall, Kenton J. Swartz, Robert J. Ferrante, and Joseph B. Martin Neurology Service, Massachusetts General Hospital, and Department of Neurology, Harvard Medical School, Boston, Massachusetts 02114 Quinolinic acid (QA) is an endogenous excitotoxin present mammalian brain, is an excitotoxin which producesaxon-spar- in mammalian brain that reproduces many of the histologic ing striatal lesions. We found that this compound produced a and neurochemical features of Huntington’s disease (HD). more exact model of HD than kainic acid, sincethe lesionswere In the present study we have examined the ability of a variety accompaniedby a relative sparingof somatostatin-neuropeptide of systemically administered compounds to modify striatal Y neurons (Beal et al., 1986a). QA neurotoxicity. Lesions were assessed by measurements If an excitotoxin is involved in the pathogenesisof HD, then of the intrinsic striatal neurotransmitters substance P, so- agentsthat modify excitotoxin lesionsin vivo could potentially matostatin, neuropeptide Y, and GABA. Histologic exami- be efficacious as therapeutic agents in HD. The best form of nation was performed with Nissl stains. The antioxidants therapy from a practical standpoint would be a drug that could ascorbic acid, beta-carotene, and alpha-tocopherol admin- be administered systemically, preferably by an oral route. In the istered S.C. for 3 d prior to striatal QA lesions had no sig- presentstudy we have therefore examined the ability of a variety nificant effect. Other drugs were administered i.p. l/2 hr prior of systemically administered drugs to modify QA striatal neu- to QA striatal lesions. -
THE ROLE of KYNURENINE, a TRYPTOPHAN METABOLITE THAT INCREASES with AGE, in MUSCLE ATROPHY and LIPID PEROXIDATION) by Helen Eliz
THE ROLE OF KYNURENINE, A TRYPTOPHAN METABOLITE THAT INCREASES WITH AGE, IN MUSCLE ATROPHY AND LIPID PEROXIDATION) By Helen Elizabeth Kaiser Submitted to the Faculty of the Graduate School of Augusta University in partial fulfillment of the Requirements of the Degree of (Doctor of Philosophy in Cellular Biology and Anatomy) April 2020 COPYRIGHT© 2020 by Helen Kaiser ACKNOWLEDGEMENTS I wish to express my deepest gratitude to my mentor Dr. Mark Hamrick. His support and advice were invaluable to my learning and development as a scientist. I would like to especially recognize Dr. Hamrick’s honesty to data, and kindness to everyone around him. It was an honor to work as his student, and to be a part of this project. I would like to thank my committee members, Drs. Carlos Isales, Meghan McGee-Lawrence, and Yutao Liu, for their continued support, guidance, and helpful suggestions. They have helped to shape and focus this project, and have augmented my experience as a student during the development of my dissertation. Additionally, I want to recognize and thank Dr. Sadanand Fulzele for sharing his wealth of knowledge in techniques, and always keeping his door open for student’s questions. Next, a most heartfelt thanks to my lab mates: Andrew Khayrullin, Bharati Mendhe, Ling Ruan and Emily Parker. This project has been a team effort, all of them have been fundamental to its success. I further want to show my appreciation for the rest of the department of Cellular Biology and Anatomy at Augusta University. The histology core members Donna Kumiski and Penny Roon for their direct help in sectioning, and contributions to this project. -
An Integrated Meta-Analysis of Peripheral Blood Metabolites and Biological Functions in Major Depressive Disorder
Molecular Psychiatry https://doi.org/10.1038/s41380-020-0645-4 ARTICLE An integrated meta-analysis of peripheral blood metabolites and biological functions in major depressive disorder 1,2,3 1,2,3 1,2,3 1,3 1,3 4,5 1,3 1,3 Juncai Pu ● Yiyun Liu ● Hanping Zhang ● Lu Tian ● Siwen Gui ● Yue Yu ● Xiang Chen ● Yue Chen ● 1,2,3 1,3 1,3 1,3 1,3 1,2,3 Lining Yang ● Yanqin Ran ● Xiaogang Zhong ● Shaohua Xu ● Xuemian Song ● Lanxiang Liu ● 1,2,3 1,3 1,2,3 Peng Zheng ● Haiyang Wang ● Peng Xie Received: 3 June 2019 / Revised: 24 December 2019 / Accepted: 10 January 2020 © The Author(s) 2020. This article is published with open access Abstract Major depressive disorder (MDD) is a serious mental illness, characterized by high morbidity, which has increased in recent decades. However, the molecular mechanisms underlying MDD remain unclear. Previous studies have identified altered metabolic profiles in peripheral tissues associated with MDD. Using curated metabolic characterization data from a large sample of MDD patients, we meta-analyzed the results of metabolites in peripheral blood. Pathway and network analyses were then performed to elucidate the biological themes within these altered metabolites. We identified 23 differentially 1234567890();,: 1234567890();,: expressed metabolites between MDD patients and controls from 46 studies. MDD patients were characterized by higher levels of asymmetric dimethylarginine, tyramine, 2-hydroxybutyric acid, phosphatidylcholine (32:1), and taurochenode- soxycholic acid and lower levels of L-acetylcarnitine, creatinine, L-asparagine, L-glutamine, linoleic acid, pyruvic acid, palmitoleic acid, L-serine, oleic acid, myo-inositol, dodecanoic acid, L-methionine, hypoxanthine, palmitic acid, L-tryptophan, kynurenic acid, taurine, and 25-hydroxyvitamin D compared with controls. -
NMDA Agonists Using ALZET Osmotic Pumps
ALZET® Bibliography References on the Administration of NMDA Agonists Using ALZET Osmotic Pumps Aspartic Acid P7453: M. Domercq, et al. Excitotoxic oligodendrocyte death and axonal damage induced by glutamate transporter inhibition. Glia 2005;52(1):36-46 ALZET Comments: Oligonucleotide, antisense; oligonucleotide sense; kainate, dihydro-; aspartic acid, DL-threo-B-benzyloxy-; Saline, sterile; CSF/CNS (optic nerve); Rabbit; 1003D; 3 days; Controls received mp w/ vehicle, or contralateral nerves; antisense (glutamate transporters GLAST + GLT-1); animal info (adult, male, white New Zealand). P6888: J. Darman, et al. Viral-induced spinal motor neuron death is non-cell-autonomous and involves glutamate excitotoxicity. Journal of Neuroscience 2004;24(34):7566-7575 ALZET Comments: Aspartic acid, dl-threo-B-hydroxy; spermine, 1-naphthyl acetyl; CSF/CNS (intrathecal, subarachnoid space); Rat; 1007D; 7 days; Controls received mp w/ saline; enzyme inhibitors (GLT-1, GluR2). P3908: A. Hirata, et al. AMPA receptor-mediated slow neuronal death in the rat spinal cord induced by long-term blockade of glutamate transporters with THA. Brain Research 1997;771(37-44 ALZET Comments: Aspartic acid, dl-threo-B-hydroxy; Glutamate, l-; CSF, artificial;; CSF/CNS (subarachnoid space, intrathecal); Rat; 2ML1; no duration posted; dose-response; cannula position verified. P0289: R. M. Mangano, et al. Chronic infusion of endogenous excitatory amino acids into rat striatum and hippocampus. Brain Res. Bull 1983;10(47-51 ALZET Comments: Aminobutyric acid, Y-; Aspartic acid, dl-threo-B-hydroxy; Aspartic acid, l-; Cysteine sulfinic acid; Glutamic acid, l-; Radio-isotopes; 3H tracer; Acetate; Saline; CSF/CNS (corpus striatum); CSF/CNS (hippocampus); Rat; 2002; 2 weeks; comparison of injec. -
Inflammation, Insulin Resistance and the Pathophysiology of Depression: Implications for Novel Antidepressant Developments
REVIEW ARTICLE Psychiatry and Clinical Psychopharmacology 2020;30(1):71-80 DOI: 10.5455/PCP.20200215032026 Inflammation, Insulin Resistance and the Pathophysiology of Depression: Implications for Novel Antidepressant Developments Brian E. Leonarda, Feyza Aricioglub a Department of Pharmacology and Therapeutics,National University of Ireland, Galway, b Department of Pharmacology and Psychopharmacology Research Unit University of Marmara, Istanbul, Turkey Abstract This review summarises the impact of chronic low grade inflammation as a causative factor in the pathophysiology of major depression. Evidence is presented to show that proinflammatory cytokines both directly, and indirectly by increasing hypercortisolaemia, desensitise insulin receptors and thereby ARTICLE HISTORY decrease the transport of glucose into the brain (the diabetic brain). In addition, the proinflammatory Received: May 30, 2019 cytokines activate the tryptophan-kynurenine pathway which results in the synthesis of the NMDA- Accepted: Jan 29, 2020 glutamate receptor agonist quinolinic acid. This acts as a neurotoxin and facilitates neuronal apoptosis. As metabolic changes initiated by low grade inflammation underlie the pathophysiology of depression, drugs which attenuate the inflammatory cascade may present an approach to the KEYWORDS: inflammation, novel development of antidepressants. The review concludes with a summary of drugs which might insulin resistance, depression be considered for their novel therapeutic activity. Key points are; i)Chronic low grade inflammation -
The Excitotoxin Quinolinic Acid Induces Tau Phosphorylation in Human Neurons
The Excitotoxin Quinolinic Acid Induces Tau Phosphorylation in Human Neurons Abdur Rahman1,4., Kaka Ting2, Karen M. Cullen3, Nady Braidy4, Bruce J. Brew2,5, Gilles J. Guillemin2,4.* 1 Department of Family Sciences, College for Women, Kuwait University, Shuwaikh, Kuwait, 2 St Vincent’s Hospital, Centre for Applied Medical Research, Department of Neuroimmunology, Darlinghurst, New South Wales, Australia, 3 Disciplines of Anatomy and Histology, School of Medical Science, The University of Sydney, New South Wales, Australia, 4 Department of Pharmacology, University of New South Wales, School of Medical Science, Sydney, New South Wales, Australia, 5 Department of Neurology, St Vincent’s Hospital, Darlinghurst, New South Wales, Australia Abstract Some of the tryptophan catabolites produced through the kynurenine pathway (KP), and more particularly the excitotoxin quinolinic acid (QA), are likely to play a role in the pathogenesis of Alzheimer’s disease (AD). We have previously shown that the KP is over activated in AD brain and that QA accumulates in amyloid plaques and within dystrophic neurons. We hypothesized that QA in pathophysiological concentrations affects tau phosphorylation. Using immunohistochemistry, we found that QA is co-localized with hyperphosphorylated tau (HPT) within cortical neurons in AD brain. We then investigated in vitro the effects of QA at various pathophysiological concentrations on tau phosphorylation in primary cultures of human neurons. Using western blot, we found that QA treatment increased the phosphorylation of tau at serine 199/202, threonine 231 and serine 396/404 in a dose dependent manner. Increased accumulation of phosphorylated tau was also confirmed by immunocytochemistry. This increase in tau phosphorylation was paralleled by a substantial decrease in the total protein phosphatase activity. -
Urinary Metabolomics to Identify a Unique Biomarker Panel for Detecting Colorectal Cancer: a Multicenter Study
Published OnlineFirst May 31, 2019; DOI: 10.1158/1055-9965.EPI-18-1291 Research Article Cancer Epidemiology, Biomarkers Urinary Metabolomics to Identify a Unique & Prevention Biomarker Panel for Detecting Colorectal Cancer: A Multicenter Study Lu Deng1, Kathleen Ismond1,2, Zhengjun Liu3, Jeremy Constable4, Haili Wang2, Olusegun I. Alatise5, Martin R. Weiser4, T.P. Kingham4, and David Chang1,2 Abstract Background: Population-based screening programs are paring the metabolomic profiles from colorectal cancer versus credited with earlier colorectal cancer diagnoses and treat- controls. Multiple models were constructed leading to a good ment initiation, which reduce mortality rates and improve separation of colorectal cancer from controls. patient health outcomes. However, recommended screen- Results: A panel of 17 metabolites was identified as possible ing methods are unsatisfactory as they are invasive, are biomarkers for colorectal cancer. Using only two of the select- resource intensive, suffer from low uptake, or have poor ed metabolites, namely diacetylspermine and kynurenine, a diagnostic performance. Our goal was to identify a urine predictor for detecting colorectal cancer was developed with an metabolomic-based biomarker panel for the detection of AUC of 0.864, a specificity of 80.0%, and a sensitivity of colorectal cancer that has the potential for global popula- 80.0%. tion-based screening. Conclusions: We present a potentially "universal" metabo- Methods: Prospective urine samples were collected from lomic biomarker panel for colorectal cancer independent of study participants. Based upon colonoscopy and histopathol- cohort clinical features based on a North American popula- ogy results, 342 participants (colorectal cancer, 171; healthy tion. Further research is needed to confirm the utility of the controls, 171) from two study sites (Canada, United States) profile in a prospective, population-based colorectal cancer were included in the analyses.