Genomic Analyses of Polysaccharide Utilization in Marine Flavobacteriia
Total Page:16
File Type:pdf, Size:1020Kb
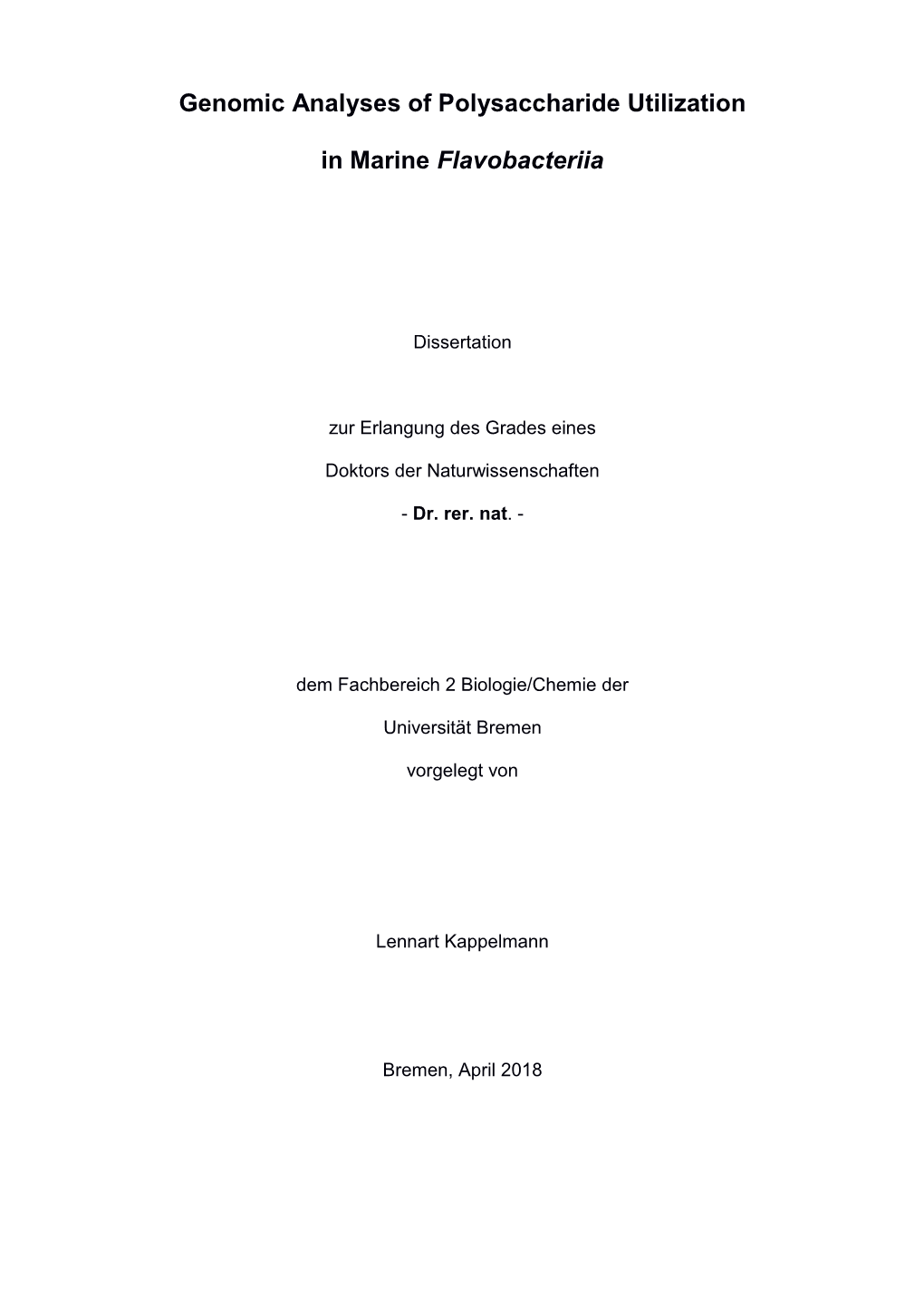
Load more
Recommended publications
-
Isabel Cristina Santos Silva De Faria Ramos Comunidade Bacteriana
Universidade de Aveiro Departamento de Biologia 2009 Isabel Cristina Santos Comunidade bacteriana cultivável da microcamada Silva de Faria Ramos superficial estuarina Culturable bacterial community of the estuarine surface microlayer Universidade de Aveiro Departamento de Biologia 2009 Isabel Cristina Santos Comunidade bacteriana cultivável da microcamada Silva de Faria Ramos superficial estuarina Culturable bacterial community of the estuarine surface microlayer dissertação apresentada à Universidade de Aveiro para cumprimento dos requisitos necessários à obtenção do grau de Mestre em Microbiologia, realizada sob a orientação científica da Prof. Dra. Isabel Henriques, Professora Auxiliar Convidada do Departamento de Biologia da Universidade de Aveiro. Dedico este trabalho à minha família por todo o apoio e compreensão. o júri presidente Prof. Doutora Sónia Alexandra Leite Velho Mendo Barroso professora auxiliar do Departamento de Biologia da Universidade de Aveiro Prof. Doutor Fernando Manuel dos Santos Tavares professor auxiliar do Departamento de Botânica, Faculdade de Ciências da Universidade do Porto Prof. Doutora Isabel da Silva Henriques professora auxiliar convidada do Departamento de Biologia da Universidade de Aveiro agradecimentos A primeira pessoa a quem quero agradecer é ao Professor António Correia pela oportunidade de desenvolver este trabalho no seu laboratório e pelo exemplo de sacrifício e constante optimismo com que temos que enfrentar a vida! Quero agradecer à minha orientadora, Doutora Isabel Henriques, com quem mantive um relação cordial e leal durante todo o trabalho, por tudo o que me ensinou…que foi muito mais além do que conhecimento científico. Aprendi a enfrentar as agruras do trabalho com perseverança e entusiasmo. A todos os meus colegas de laboratório com quem convivi e partilhei todas as minhas alegrias e frustrações. -
New Zealand's Genetic Diversity
1.13 NEW ZEALAND’S GENETIC DIVERSITY NEW ZEALAND’S GENETIC DIVERSITY Dennis P. Gordon National Institute of Water and Atmospheric Research, Private Bag 14901, Kilbirnie, Wellington 6022, New Zealand ABSTRACT: The known genetic diversity represented by the New Zealand biota is reviewed and summarised, largely based on a recently published New Zealand inventory of biodiversity. All kingdoms and eukaryote phyla are covered, updated to refl ect the latest phylogenetic view of Eukaryota. The total known biota comprises a nominal 57 406 species (c. 48 640 described). Subtraction of the 4889 naturalised-alien species gives a biota of 52 517 native species. A minimum (the status of a number of the unnamed species is uncertain) of 27 380 (52%) of these species are endemic (cf. 26% for Fungi, 38% for all marine species, 46% for marine Animalia, 68% for all Animalia, 78% for vascular plants and 91% for terrestrial Animalia). In passing, examples are given both of the roles of the major taxa in providing ecosystem services and of the use of genetic resources in the New Zealand economy. Key words: Animalia, Chromista, freshwater, Fungi, genetic diversity, marine, New Zealand, Prokaryota, Protozoa, terrestrial. INTRODUCTION Article 10b of the CBD calls for signatories to ‘Adopt The original brief for this chapter was to review New Zealand’s measures relating to the use of biological resources [i.e. genetic genetic resources. The OECD defi nition of genetic resources resources] to avoid or minimize adverse impacts on biological is ‘genetic material of plants, animals or micro-organisms of diversity [e.g. genetic diversity]’ (my parentheses). -
CUED Phd and Mphil Thesis Classes
High-throughput Experimental and Computational Studies of Bacterial Evolution Lars Barquist Queens' College University of Cambridge A thesis submitted for the degree of Doctor of Philosophy 23 August 2013 Arrakis teaches the attitude of the knife { chopping off what's incomplete and saying: \Now it's complete because it's ended here." Collected Sayings of Muad'dib Declaration High-throughput Experimental and Computational Studies of Bacterial Evolution The work presented in this dissertation was carried out at the Wellcome Trust Sanger Institute between October 2009 and August 2013. This dissertation is the result of my own work and includes nothing which is the outcome of work done in collaboration except where specifically indicated in the text. This dissertation does not exceed the limit of 60,000 words as specified by the Faculty of Biology Degree Committee. This dissertation has been typeset in 12pt Computer Modern font using LATEX according to the specifications set by the Board of Graduate Studies and the Faculty of Biology Degree Committee. No part of this dissertation or anything substantially similar has been or is being submitted for any other qualification at any other university. Acknowledgements I have been tremendously fortunate to spend the past four years on the Wellcome Trust Genome Campus at the Sanger Institute and the European Bioinformatics Institute. I would like to thank foremost my main collaborators on the studies described in this thesis: Paul Gardner and Gemma Langridge. Their contributions and support have been invaluable. I would also like to thank my supervisor, Alex Bateman, for giving me the freedom to pursue a wide range of projects during my time in his group and for advice. -
Eelgrass Sediment Microbiome As a Nitrous Oxide Sink in Brackish Lake Akkeshi, Japan
Microbes Environ. Vol. 34, No. 1, 13-22, 2019 https://www.jstage.jst.go.jp/browse/jsme2 doi:10.1264/jsme2.ME18103 Eelgrass Sediment Microbiome as a Nitrous Oxide Sink in Brackish Lake Akkeshi, Japan TATSUNORI NAKAGAWA1*, YUKI TSUCHIYA1, SHINGO UEDA1, MANABU FUKUI2, and REIJI TAKAHASHI1 1College of Bioresource Sciences, Nihon University, 1866 Kameino, Fujisawa, 252–0880, Japan; and 2Institute of Low Temperature Science, Hokkaido University, Kita-19, Nishi-8, Kita-ku, Sapporo, 060–0819, Japan (Received July 16, 2018—Accepted October 22, 2018—Published online December 1, 2018) Nitrous oxide (N2O) is a powerful greenhouse gas; however, limited information is currently available on the microbiomes involved in its sink and source in seagrass meadow sediments. Using laboratory incubations, a quantitative PCR (qPCR) analysis of N2O reductase (nosZ) and ammonia monooxygenase subunit A (amoA) genes, and a metagenome analysis based on the nosZ gene, we investigated the abundance of N2O-reducing microorganisms and ammonia-oxidizing prokaryotes as well as the community compositions of N2O-reducing microorganisms in in situ and cultivated sediments in the non-eelgrass and eelgrass zones of Lake Akkeshi, Japan. Laboratory incubations showed that N2O was reduced by eelgrass sediments and emitted by non-eelgrass sediments. qPCR analyses revealed that the abundance of nosZ gene clade II in both sediments before and after the incubation as higher in the eelgrass zone than in the non-eelgrass zone. In contrast, the abundance of ammonia-oxidizing archaeal amoA genes increased after incubations in the non-eelgrass zone only. Metagenome analyses of nosZ genes revealed that the lineages Dechloromonas-Magnetospirillum-Thiocapsa and Bacteroidetes (Flavobacteriia) within nosZ gene clade II were the main populations in the N2O-reducing microbiome in the in situ sediments of eelgrass zones. -
Spatiotemporal Dynamics of Marine Bacterial and Archaeal Communities in Surface Waters Off the Northern Antarctic Peninsula
Spatiotemporal dynamics of marine bacterial and archaeal communities in surface waters off the northern Antarctic Peninsula Camila N. Signori, Vivian H. Pellizari, Alex Enrich Prast and Stefan M. Sievert The self-archived postprint version of this journal article is available at Linköping University Institutional Repository (DiVA): http://urn.kb.se/resolve?urn=urn:nbn:se:liu:diva-149885 N.B.: When citing this work, cite the original publication. Signori, C. N., Pellizari, V. H., Enrich Prast, A., Sievert, S. M., (2018), Spatiotemporal dynamics of marine bacterial and archaeal communities in surface waters off the northern Antarctic Peninsula, Deep-sea research. Part II, Topical studies in oceanography, 149, 150-160. https://doi.org/10.1016/j.dsr2.2017.12.017 Original publication available at: https://doi.org/10.1016/j.dsr2.2017.12.017 Copyright: Elsevier http://www.elsevier.com/ Spatiotemporal dynamics of marine bacterial and archaeal communities in surface waters off the northern Antarctic Peninsula Camila N. Signori1*, Vivian H. Pellizari1, Alex Enrich-Prast2,3, Stefan M. Sievert4* 1 Departamento de Oceanografia Biológica, Instituto Oceanográfico, Universidade de São Paulo (USP). Praça do Oceanográfico, 191. CEP: 05508-900 São Paulo, SP, Brazil. 2 Department of Thematic Studies - Environmental Change, Linköping University. 581 83 Linköping, Sweden 3 Departamento de Botânica, Instituto de Biologia, Universidade Federal do Rio de Janeiro (UFRJ). Av. Carlos Chagas Filho, 373. CEP: 21941-902. Rio de Janeiro, Brazil 4 Biology Department, Woods Hole Oceanographic Institution (WHOI). 266 Woods Hole Road, Woods Hole, MA 02543, United States. *Corresponding authors: Camila Negrão Signori Address: Departamento de Oceanografia Biológica, Instituto Oceanográfico, Universidade de São Paulo, São Paulo, Brazil. -
Leeuwenhoekiella Palythoae Sp. Nov., a New Member of the Family Flavobacteriaceae
International Journal of Systematic and Evolutionary Microbiology (2009), 59, 3074–3077 DOI 10.1099/ijs.0.010371-0 Leeuwenhoekiella palythoae sp. nov., a new member of the family Flavobacteriaceae Olga I. Nedashkovskaya,1 Marc Vancanneyt,2 Seung Bum Kim,3 Natalia V. Zhukova,4 Ji Hye Han3 and Valery V. Mikhailov1 Correspondence 1Pacific Institute of Bioorganic Chemistry of the Far-Eastern Branch of the Russian Academy of Olga I. Nedashkovskaya Sciences, Pr. 100 Let Vladivostoku 159, 690022 Vladivostok, Russia [email protected] 2BCCM/LMG Bacteria Collection, and Laboratory of Microbiology, Ghent University, Ledeganckstraat 35, B-9000 Ghent, Belgium 3Department of Microbiology, School of Bioscience and Biotechnology, Chungnam National University, 220 Gung-dong, Yuseong, Daejeon 305-764, Republic of Korea 4Institute of Marine Biology of the Far-Eastern Branch of the Russian Academy of Sciences, Pal’chevskogo St. 17, 690032, Vladivostok, Russia The taxonomic status of a novel, heterotrophic, strictly aerobic, gliding and yellow–orange- pigmented bacterium (strain KMM 6264T), associated with the coral Palythoa, was determined. The 16S rRNA gene sequence analysis indicated that strain KMM 6264T clustered with the recognized species of the genus Leeuwenhoekiella of the family Flavobacteriaceae with 96.4– 98.2 % sequence similarity. DNA–DNA reassociation levels between the isolate and the type strains of Leeuwenhoekiella species were 15–22 %. The DNA G+C content was 41.2 mol%. The phylogenetic evidence and the results of genomic and phenotypic analyses showed that the isolate should be classified as a member of a novel species of the genus Leeuwenhoekiella, for which the name Leeuwenhoekiella palythoae sp. nov. -
Host-Parasite Interaction of Atlantic Salmon (Salmo Salar) and the Ectoparasite Neoparamoeba Perurans in Amoebic Gill Disease
ORIGINAL RESEARCH published: 31 May 2021 doi: 10.3389/fimmu.2021.672700 Host-Parasite Interaction of Atlantic salmon (Salmo salar) and the Ectoparasite Neoparamoeba perurans in Amoebic Gill Disease † Natasha A. Botwright 1*, Amin R. Mohamed 1 , Joel Slinger 2, Paula C. Lima 1 and James W. Wynne 3 1 Livestock and Aquaculture, CSIRO Agriculture and Food, St Lucia, QLD, Australia, 2 Livestock and Aquaculture, CSIRO Agriculture and Food, Woorim, QLD, Australia, 3 Livestock and Aquaculture, CSIRO Agriculture and Food, Hobart, TAS, Australia Marine farmed Atlantic salmon (Salmo salar) are susceptible to recurrent amoebic gill disease Edited by: (AGD) caused by the ectoparasite Neoparamoeba perurans over the growout production Samuel A. M. Martin, University of Aberdeen, cycle. The parasite elicits a highly localized response within the gill epithelium resulting in United Kingdom multifocal mucoid patches at the site of parasite attachment. This host-parasite response Reviewed by: drives a complex immune reaction, which remains poorly understood. To generate a model Diego Robledo, for host-parasite interaction during pathogenesis of AGD in Atlantic salmon the local (gill) and University of Edinburgh, United Kingdom systemic transcriptomic response in the host, and the parasite during AGD pathogenesis was Maria K. Dahle, explored. A dual RNA-seq approach together with differential gene expression and system- Norwegian Veterinary Institute (NVI), Norway wide statistical analyses of gene and transcription factor networks was employed. A multi- *Correspondence: tissue transcriptomic data set was generated from the gill (including both lesioned and non- Natasha A. Botwright lesioned tissue), head kidney and spleen tissues naïve and AGD-affected Atlantic salmon [email protected] sourced from an in vivo AGD challenge trial. -
Recurring Patterns in Bacterioplankton Dynamics During Coastal Spring
RESEARCH ARTICLE Recurring patterns in bacterioplankton dynamics during coastal spring algae blooms Hanno Teeling1*†, Bernhard M Fuchs1*†, Christin M Bennke1‡, Karen Kru¨ ger1, Meghan Chafee1, Lennart Kappelmann1, Greta Reintjes1, Jost Waldmann1, Christian Quast1, Frank Oliver Glo¨ ckner1, Judith Lucas2, Antje Wichels2, Gunnar Gerdts2, Karen H Wiltshire3, Rudolf I Amann1* 1Max Planck Institute for Marine Microbiology, Bremen, Germany; 2Biologische Anstalt Helgoland, Alfred Wegener Institute for Polar and Marine Research, Helgoland, Germany; 3Alfred Wegener Institute for Polar and Marine Research, List auf Sylt, Germany Abstract A process of global importance in carbon cycling is the remineralization of algae biomass by heterotrophic bacteria, most notably during massive marine algae blooms. Such blooms can trigger secondary blooms of planktonic bacteria that consist of swift successions of distinct *For correspondence: hteeling@ mpi-bremen.de (HT); bfuchs@mpi- bacterial clades, most prominently members of the Flavobacteriia, Gammaproteobacteria and the bremen.de (BMF); ramann@mpi- alphaproteobacterial Roseobacter clade. We investigated such successions during spring bremen.de (RIA) phytoplankton blooms in the southern North Sea (German Bight) for four consecutive years. Dense sampling and high-resolution taxonomic analyses allowed the detection of recurring patterns down † These authors contributed to the genus level. Metagenome analyses also revealed recurrent patterns at the functional level, in equally to this work particular with respect to algal polysaccharide degradation genes. We, therefore, hypothesize that Present address: ‡Section even though there is substantial inter-annual variation between spring phytoplankton blooms, the Biology, Leibniz Institute for accompanying succession of bacterial clades is largely governed by deterministic principles such as Baltic Sea Research, substrate-induced forcing. -
Cochleicola Gelatinilyticus Gen. Nov., Sp. Nov., Isolated from a Marine Gastropod, Reichia Luteostoma S Su-Kyoung Shin1, Eunji Kim1, Sungmi Choi1, and Hana Yi1,2*
J. Microbiol. Biotechnol. (2016), 26(8), 1439–1445 http://dx.doi.org/10.4014/jmb.1604.04083 Research Article Review jmb Cochleicola gelatinilyticus gen. nov., sp. nov., Isolated from a Marine Gastropod, Reichia luteostoma S Su-Kyoung Shin1, Eunji Kim1, Sungmi Choi1, and Hana Yi1,2* 1BK21PLUS Program in Embodiment: Health-Society Interaction, Department of Public Health Sciences, Graduate School, Korea University, Seoul 02841, Republic of Korea 2School of Biosystem and Biomedical Science, Department of Public Health Science, Korea University, Seoul 02841, Republic of Korea Received: April 29, 2016 Revised: May 13, 2016 A yellow, rod-shaped, non-motile, gram-negative, and strictly aerobic bacterial strain, Accepted: May 18, 2016 designated LPB0005T, was isolated from a marine gastropod, Reichia luteostoma. Here the genome sequence was determined, which comprised 3,395,737 bp with 2,962 protein-coding First published online genes. The DNA G+C content was 36.3 mol%. The 16S rRNA gene sequence analysis indicated May 20, 2016 that the isolate represents a novel genus and species in the family Flavobacteriaceae, with *Corresponding author relatively low sequence similarities to other closely related genera. The isolate showed Phone: +82-2-3290-5644; chemotaxonomic properties within the range reported for the family Flavobacteriaceae, but E-mail: [email protected] possesses many physiological and biochemical characteristics that distinguished it from S upplementary data for this species in the closely related genera Ulvibacter, Jejudonia, and Aureitalea. Based on paper are available on-line only at phylogenetic, phenotypic, and genomic analyses, strain LPB0005T represents a novel genus http://jmb.or.kr. and species, for which the name Cochleicola gelatinilyticus gen. -
Targeting of Flavobacterium Johnsoniae Proteins for Secretion by the Type IX Secretion System Surashree Sunil Kulkarni University of Wisconsin-Milwaukee
University of Wisconsin Milwaukee UWM Digital Commons Theses and Dissertations May 2017 Targeting of Flavobacterium Johnsoniae Proteins for Secretion By the Type IX Secretion System Surashree Sunil Kulkarni University of Wisconsin-Milwaukee Follow this and additional works at: https://dc.uwm.edu/etd Part of the Microbiology Commons Recommended Citation Kulkarni, Surashree Sunil, "Targeting of Flavobacterium Johnsoniae Proteins for Secretion By the Type IX Secretion System" (2017). Theses and Dissertations. 1501. https://dc.uwm.edu/etd/1501 This Dissertation is brought to you for free and open access by UWM Digital Commons. It has been accepted for inclusion in Theses and Dissertations by an authorized administrator of UWM Digital Commons. For more information, please contact [email protected]. TARGETING OF FLAVOBACTERIUM JOHNSONIAE PROTEINS FOR SECRETION BY THE TYPE IX SECRETION SYSTEM by Surashree S. Kulkarni A Dissertation Submitted in Partial Fulfillment of the Requirements for the Degree of Doctor of Philosophy in Biological Sciences at The University of Wisconsin-Milwaukee May 2017 ABSTRACT TARGETING OF FLAVOBACTERIUM JOHNSONIAE PROTEINS FOR SECRETION BY THE TYPE IX SECRETION SYSTEM by Surashree S. Kulkarni The University of Wisconsin-Milwaukee, 2017 Under the Supervision of Dr. Mark J. McBride Flavobacterium johnsoniae and many related bacteria secrete proteins across the outer membrane using the type IX secretion system (T9SS). Proteins secreted by T9SSs have amino-terminal signal peptides for export across the cytoplasmic membrane by the Sec system and carboxy-terminal domains (CTDs) targeting them for secretion across the outer membrane by the T9SS. Most but not all T9SS CTDs belong to family TIGR04183 (type A CTDs). -
Tenacibaculum Maritimum, Causal Agent of Tenacibaculosis in Marine Fish
# 70 JANUARY 2019 Tenacibaculum maritimum, causal agent of tenacibaculosis in marine fish ICES IDENTIFICATION LEAFLETS FOR DISEASES AND PARASITES IN FISH AND SHELLFISH ICES INTERNATIONAL COUNCIL FOR THE EXPLORATION OF THE SEA CIEM CONSEIL INTERNATIONAL POUR L’EXPLORATION DE LA MER ICES IDENTIFICATION LEAFLETS FOR DISEASES AND PARASITES OF FISH AND SHELLFISH NO. 70 JANUARY 2019 Tenacibaculum maritimum, causal agent of tenacibaculosis in marine fish Original by Y. Santos, F. Pazos and J. L. Barja (No. 55) Revised by Simon R. M. Jones and Lone Madsen International Council for the Exploration of the Sea Conseil International pour l’Exploration de la Mer H. C. Andersens Boulevard 44–46 DK-1553 Copenhagen V Denmark Telephone (+45) 33 38 67 00 Telefax (+45) 33 93 42 15 www.ices.dk [email protected] Recommended format for purposes of citation: ICES 2019. Tenacibaculum maritimum, causal agent of tenacibaculosis in marine fish. Orig- inal by Santos Y., F. Pazos and J. L. Barja (No. 55), Revised by Simon R. M. Jones and Lone Madsen. ICES Identification Leaflets for Diseases and Parasites of Fish and Shell- fish. No. 70. 5 pp. http://doi.org/10.17895/ices.pub.4681 Series Editor: Neil Ruane. Prepared under the auspices of the ICES Working Group on Pathology and Diseases of Marine Organisms. The material in this report may be reused for non-commercial purposes using the rec- ommended citation. ICES may only grant usage rights of information, data, images, graphs, etc. of which it has ownership. For other third-party material cited in this re- port, you must contact the original copyright holder for permission. -
First Isolation of Virulent Tenacibaculum Maritimum Strains
bioRxiv preprint doi: https://doi.org/10.1101/2021.03.15.435441; this version posted March 15, 2021. The copyright holder for this preprint (which was not certified by peer review) is the author/funder, who has granted bioRxiv a license to display the preprint in perpetuity. It is made available under aCC-BY 4.0 International license. 1 First isolation of virulent Tenacibaculum maritimum 2 strains from diseased orbicular batfish (Platax orbicularis) 3 farmed in Tahiti Island 4 Pierre Lopez 1¶, Denis Saulnier 1¶*, Shital Swarup-Gaucher 2, Rarahu David 2, Christophe Lau 2, 5 Revahere Taputuarai 2, Corinne Belliard 1, Caline Basset 1, Victor Labrune 1, Arnaud Marie 3, Jean 6 François Bernardet 4, Eric Duchaud 4 7 8 9 1 Ifremer, IRD, Institut Louis‐Malardé, Univ Polynésie française, EIO, Labex Corail, F‐98719 10 Taravao, Tahiti, Polynésie française, France 11 2 DRM, Direction des ressources marines, Fare Ute Immeuble Le caill, BP 20 – 98713 Papeete, Tahiti, 12 Polynésie française 13 3 Labofarm Finalab Veterinary Laboratory Group, 4 rue Théodore Botrel, 22600 Loudéac, France 14 4 Unité VIM, INRAE, Université Paris-Saclay, 78350 Jouy-en-Josas, France 15 * Corresponding author 16 E-mail: [email protected] 1 bioRxiv preprint doi: https://doi.org/10.1101/2021.03.15.435441; this version posted March 15, 2021. The copyright holder for this preprint (which was not certified by peer review) is the author/funder, who has granted bioRxiv a license to display the preprint in perpetuity. It is made available under aCC-BY 4.0 International license. 17 Abstract 18 The orbicular batfish (Platax orbicularis), also called 'Paraha peue' in Tahitian, is the most important 19 marine fish species reared in French Polynesia.