Food-Derived Compounds Apigenin and Luteolin Modulate Mrna Splicing of Introns with Weak Splice Sites
Total Page:16
File Type:pdf, Size:1020Kb
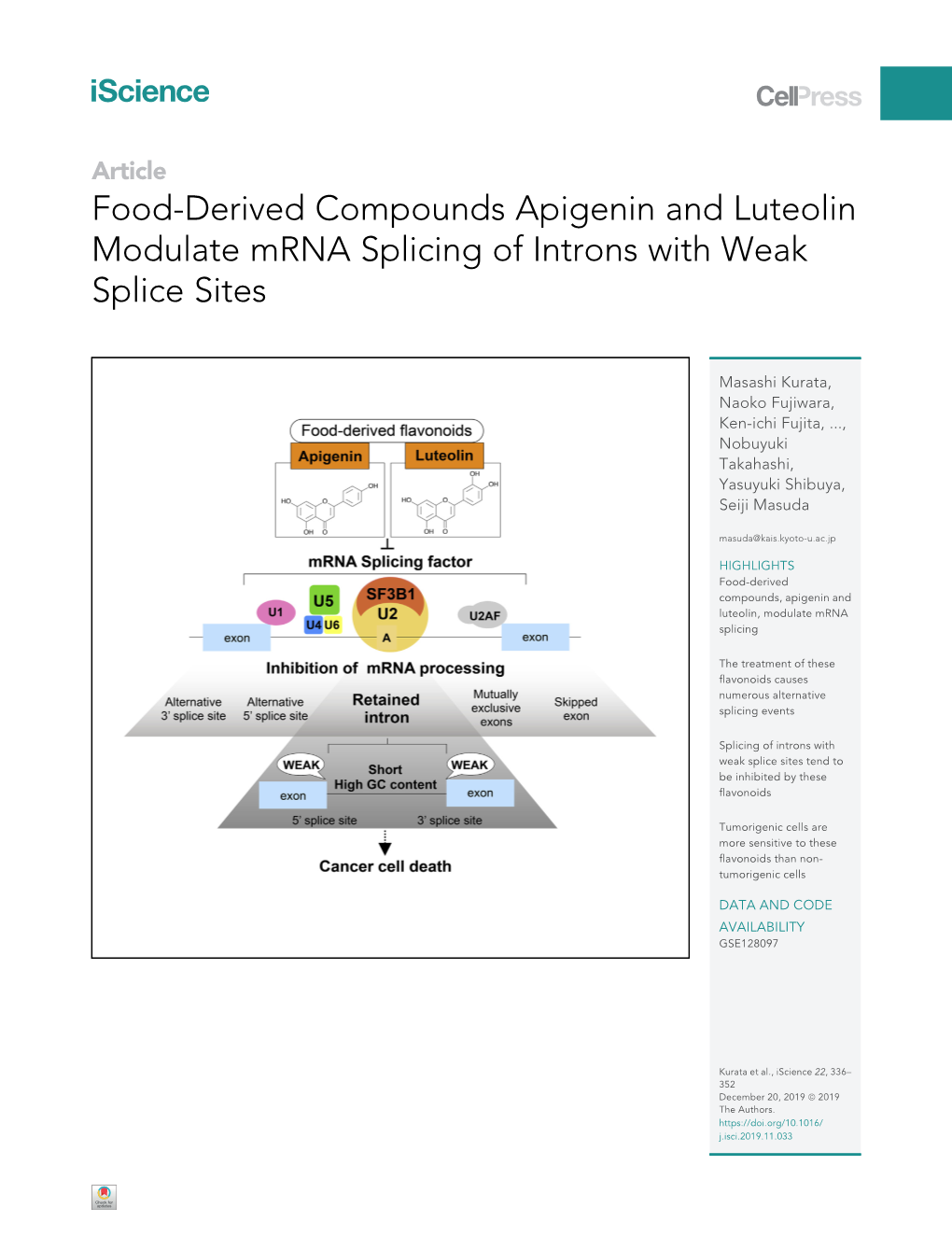
Load more
Recommended publications
-
Curcumin, EGCG, Resveratrol and Quercetin on Flying Carpets
DOI:http://dx.doi.org/10.7314/APJCP.2014.15.9.3865 Targeting Cancer with Nano-Bullets: Curcumin, EGCG, Resveratrol and Quercetin on Flying Carpets MINI-REVIEW Targeting Cancer with Nano-Bullets: Curcumin, EGCG, Resveratrol and Quercetin on Flying Carpets Aliye Aras1, Abdur Rehman Khokhar2, Muhammad Zahid Qureshi3, Marcela Fernandes Silva4, Agnieszka Sobczak-Kupiec5, Edgardo Alfonso Gómez Pineda4, Ana Adelina Winkler Hechenleitner4, Ammad Ahmad Farooqi6* Abstract It is becoming progressively more understandable that different phytochemicals isolated from edible plants interfere with specific stages of carcinogenesis. Cancer cells have evolved hallmark mechanisms to escape from death. Concordant with this approach, there is a disruption of spatiotemproal behaviour of signaling cascades in cancer cells, which can escape from apoptosis because of downregulation of tumor suppressor genes and over- expression of oncogenes. Genomic instability, intra-tumor heterogeneity, cellular plasticity and metastasizing potential of cancer cells all are related to molecular alterations. Data obtained through in vitro studies has convincingly revealed that curcumin, EGCG, resveratrol and quercetin are promising anticancer agents. Their efficacy has been tested in tumor xenografted mice and considerable experimental findings have stimulated researchers to further improve the bioavailability of these nutraceuticals. We partition this review into different sections with emphasis on how bioavailability of curcumin, EGCG, resveratrol and quercetin has improved using different nanotechnology approaches. Keywords: Resveratrol - nanotechnology - EGCG - apoptosis Asian Pac J Cancer Prev, 15 (9), 3865-3871 Introduction Huixiao et al., 2012) Nanoparticles have wide ranging applications because of specific properties resulting Preclinical and clinical studies have shown that cancer from both high specific surface and quantum limitation is a genomically complex disease. -
Polyphenols As Possible Agents for Pancreatic Diseases
antioxidants Review Polyphenols as Possible Agents for Pancreatic Diseases Uroš Gaši´c 1 , Ivanka Ciri´c´ 2 , Tomislav Pejˇci´c 3, Dejan Radenkovi´c 4,5, Vladimir Djordjevi´c 5, Siniša Radulovi´c 6 and Živoslav Teši´c 7,* 1 Department of Plant Physiology, Institute for Biological Research “Siniša Stankovi´c”,National Institute of Republic of Serbia, University of Belgrade, Bulevar Despota Stefana 142, 11060 Belgrade, Serbia; [email protected] 2 Innovation Center, University of Belgrade—Faculty of Chemistry, P.O. Box 51, 11158 Belgrade, Serbia; [email protected] 3 Clinic of Urology, Clinical Centre of Serbia, Pasterova 2, 11000 Belgrade, Serbia; [email protected] 4 University of Belgrade—Faculty of Medicine, dr Suboti´ca8, 11000 Belgrade, Serbia; [email protected] 5 First Surgical Clinic, Clinical Center of Serbia, Koste Todorovi´ca6, 11000 Belgrade, Serbia; [email protected] 6 Institute for Oncology and Radiology of Serbia, Pasterova 14, 11000 Belgrade, Serbia; [email protected] 7 University of Belgrade—Faculty of Chemistry, Studentski trg 12–16, P.O. Box 51, 11158 Belgrade, Serbia * Correspondence: [email protected]; Tel.: +381-113336733 Received: 29 April 2020; Accepted: 31 May 2020; Published: 23 June 2020 Abstract: Pancreatic cancer (PC) is very aggressive and it is estimated that it kills nearly 50% of patients within the first six months. The lack of symptoms specific to this disease prevents early diagnosis and treatment. Today, gemcitabine alone or in combination with other cytostatic agents such as cisplatin (Cis), 5-fluorouracil (5-FU), irinotecan, capecitabine, or oxaliplatin (Oxa) is used in conventional therapy. -
Potential Adverse Effects of Resveratrol: a Literature Review
International Journal of Molecular Sciences Review Potential Adverse Effects of Resveratrol: A Literature Review Abdullah Shaito 1 , Anna Maria Posadino 2, Nadin Younes 3, Hiba Hasan 4 , Sarah Halabi 5, Dalal Alhababi 3, Anjud Al-Mohannadi 3, Wael M Abdel-Rahman 6 , Ali H. Eid 7,*, Gheyath K. Nasrallah 3,* and Gianfranco Pintus 6,2,* 1 Department of Biological and Chemical Sciences, Lebanese International University, 1105 Beirut, Lebanon; [email protected] 2 Department of Biomedical Sciences, University of Sassari, 07100 Sassari, Italy; [email protected] 3 Department of Biomedical Science, College of Health Sciences, and Biomedical Research Center Qatar University, P.O Box 2713 Doha, Qatar; [email protected] (N.Y.); [email protected] (D.A.); [email protected] (A.A.-M.) 4 Institute of Anatomy and Cell Biology, Justus-Liebig-University Giessen, 35392 Giessen, Germany; [email protected] 5 Biology Department, Faculty of Arts and Sciences, American University of Beirut, 1105 Beirut, Lebanon; [email protected] 6 Department of Medical Laboratory Sciences, College of Health Sciences and Sharjah Institute for Medical Research, University of Sharjah, Sharjah P.O Box: 27272, United Arab Emirates; [email protected] 7 Department of Pharmacology and Toxicology, Faculty of Medicine, American University of Beirut, P.O. Box 11-0236 Beirut, Lebanon * Correspondence: [email protected] (A.H.E.); [email protected] (G.K.N.); [email protected] (G.P.) Received: 13 December 2019; Accepted: 15 March 2020; Published: 18 March 2020 Abstract: Due to its health benefits, resveratrol (RE) is one of the most researched natural polyphenols. -
Suppression of Prostate Cancer Growth by Resveratrol in The
Prostate Cancer Chemoprevention by Resveratrol RESEARCH COMMUNICATION Suppression of Prostate Cancer Growth by Resveratrol in The Transgenic Rat for Adenocarcinoma of Prostate (TRAP) Model Azman Seeni, Satoru Takahashi*, Kentaro Takeshita, Mingxi Tang, Satoshi Sugiura, Shin-ya Sato, Tomoyuki Shirai Abstract Research into actions of resveratrol, abundantly present in red grape skin, has been greatly stimulated by its reported beneficial health influence. Since it was recently proposed as a potential prostate cancer chemopreventive agent, we here performed an in vivo experiment to explore its effect in the Transgenic Rat for Adenocarcinoma of Prostate (TRAP) model, featuring the rat probasin promoter/SV 40 T antigen. Resveratrol suppressed prostate cancer growth and induction of apoptosis through androgen receptor (AR) down-regulation, without any sign of toxicity. Resveratrol not only downregulated androgen receptor (AR) expression but also suppressed the androgen responsive glandular kallikrein 11 (Gk11), known to be an ortholog of the human prostate specific antigen (PSA), at the mRNA level. The data provide a mechanistic basis for resveratrol chemopreventive efficacy against prostate cancer. Key Words: Chemoprevention - prostate cancer - resveratrol - TRAP rats -Asian Pacific J Cancer Prev, 9, 7-14 Introduction ideal tool to gain insights into possible mechanisms for prostate cancer prevention (Asamoto et al., 2001b; Cho Prostate cancer has become the most frequently et al., 2003; Zeng et al., 2005; Kandori et al., 2005; Said diagnosed cancer and the second leading cause of cancer- et al., 2006; Tang et al., 2007) in the relatively short-term. related death for men in the United States (Jemal et al., To our knowledge, the present study provided the first 2007). -
Effects on Systemic Sex Steroid Hormone
Chow et al. Journal of Translational Medicine 2014, 12:223 http://www.translational-medicine.com/content/12/1/223 RESEARCH Open Access A pilot clinical study of resveratrol in postmenopausal women with high body mass index: effects on systemic sex steroid hormones H-H Sherry Chow1*, Linda L Garland1, Brandy M Heckman-Stoddard2, Chiu-Hsieh Hsu1, Valerie D Butler1, Catherine A Cordova1, Wade M Chew1 and Terri L Cornelison2 Abstract Background: Breast cancer risk is partially determined by several hormone-related factors. Preclinical and clinical studies suggested that resveratrol may modulate these hormonal factors. Methods: We conducted a pilot study in postmenopausal women with high body mass index (BMI ≥ 25 kg/m2)to determine the clinical effect of resveratrol on systemic sex steroid hormones. Forty subjects initiated the resveratrol intervention (1 gm daily for 12 weeks) with six withdrawn early due to adverse events (AEs). Thirty-four subjects completed the intervention. Results: Resveratrol intervention did not result in significant changes in serum concentrations of estradiol, estrone, and testosterone but led to an average of 10% increase in the concentrations of sex steroid hormone binding globulin (SHBG). Resveratrol intervention resulted in an average of 73% increase in urinary 2-hydroxyestrone (2-OHE1) levels leading to a favorable change in urinary 2-OHE1/16α-OHE1 ratio. One participant had asymptomatic Grade 4 elevation of liver enzymes at the end of study intervention. Two subjects had Grade 3 skin rashes. The remaining adverse events were Grade 1 or 2 events. The most common adverse events were diarrhea and increased total cholesterol, reported in 30% and 27.5% of the subjects, respectively. -
Hydroxytyrosol but Not Resveratrol Ingestion Induced an Acute Increment of Post Exercise Blood Flow in Brachial Artery
Health, 2016, 8, 1766-1777 http://www.scirp.org/journal/health ISSN Online: 1949-5005 ISSN Print: 1949-4998 Hydroxytyrosol But Not Resveratrol Ingestion Induced an Acute Increment of Post Exercise Blood Flow in Brachial Artery Giorgia Sarais1, Antonio Crisafulli2, Daniele Concu3, Andrea Fois4, Abdallah Raweh5, Alberto Concu3,5 1Department of Life and Environmental Sciences, University of Cagliari, Cagliari, Italy 2Laboratory of Sports Physiology, University of Cagliari, Cagliari, Italy 3IIC Technologies Ltd., Cagliari, Italy 4EventFeel Ltd., Cagliari, Italy 5Medical Sciences Faculty, The LUdeS Foundation Higher Education Institution, Kalkara, Malta How to cite this paper: Sarais, G., Crisaful- Abstract li, A., Concu, D., Fois, A., Raweh, A. and Concu, A. (2016) Hydroxytyrosol But Not The aim of this study was to test if previous ingestion of compounds containing res- Resveratrol Ingestion Induced an Acute veratrol or hydroxytyrosol, followed by an exhausting hand grip exercise, could in- Increment of Post Exercise Blood Flow in duce an acute post-exercise increase in brachial blood flow. Six healthy subjects Brachial Artery. Health, 8, 1766-1777. http://dx.doi.org/10.4236/health.2016.815170 (three males and three females, 35 ± 7 years), 60 minutes after ingestion of a capsule containing 200 mg of resveratrol or 30 ml of extra virgin olive oil enriched with ty- Received: August 19, 2016 rosol, oleuropein and hydroxytyrosol, performed a hand grip exercise equal to half of Accepted: December 11, 2016 their maximum strength until they were no longer able to express the same force Published: December 14, 2016 (2-day interval between tests). The nonparametric Wilcoxon signed rank test was Copyright © 2016 by authors and used for statistical evaluations. -
Biofactors in Food Promote Health by Enhancing Mitochondrial Function
ReVieW aRticle ▼ Biofactors in food promote health by enhancing mitochondrial function by Sonia F. Shenoy, Winyoo Chowanadisai, Edward Sharman, Carl L. Keen, Jiankang Liu and Robert B. Rucker Mitochondrial function has been linked to protection from and symptom reduc- UC Davis M. Steinberg, Francine tion in chronic diseases such as heart dis- ease, diabetes and metabolic syndrome. We review a number of phytochemicals and biofactors that influence mitochon- drial function and oxidative metabolism. These include resveratrol found in grapes; several plant-derived flavonoids (quercetin, epicatechin, catechin and procyanidins); and two tyrosine-derived quinones, hydroxytyrosol in olive oil and pyrroloquinoline quinone, a minor but ubiquitous component of plant and animal tissues. In plants, these biofac- tors serve as pigments, phytoalexins or growth factors. In animals, positive nutritional and physiological attributes Biofactors in food play a role in enhancing mitochondrial function, thereby decreasing the risk of some chronic diseases. Top, a mouse that has been deprived of pyrroloquinoline quinone (PQQ), have been established for each, particu- a ubiquitous bacterial compound found in fermented products, tea, cocoa and legumes. Above, a larly with respect to their ability to affect mouse fed a diet containing PQQ. energy metabolism, cell signaling and mitochondrial function. that our body does not normally produce) attributes has been described and vali- that must be either eliminated or put to dated for each of these compounds. novel uses in the body. Many xenobiotics Biological properties of resveratrol ne of the most promising current ar- in foods can influence specific metabolic Oeas of nutritional research focuses on functions, acting as bioactive factors (bio- Resveratrol is a stilbenoid (a type of plant compounds with positive health ef- factors). -
Prevention by Dietary Polyphenols (Resveratrol, Quercetin, Apigenin)
cells Article Prevention by Dietary Polyphenols (Resveratrol, Quercetin, Apigenin) Against 7-Ketocholesterol-Induced Oxiapoptophagy in Neuronal N2a Cells: Potential Interest for the Treatment of Neurodegenerative and Age-Related Diseases Aline Yammine 1,2, Amira Zarrouk 3,4, Thomas Nury 1 , Anne Vejux 1 , Norbert Latruffe 1 , Dominique Vervandier-Fasseur 5 , Mohammad Samadi 6, John J. Mackrill 7 , Hélène Greige-Gerges 2, Lizette Auezova 2 and Gérard Lizard 1,* 1 Team Bio-peroxIL, “Biochemistry of the Peroxisome, Inflammation and Lipid Metabolism” (EA7270), University Bourgogne Franche-Comté, Inserm, 21000 Dijon, France; [email protected] (A.Y.); [email protected] (T.N.); [email protected] (A.V.); norbert.latruff[email protected] (N.L.) 2 Bioactive Molecules Research Laboratory, Doctoral School of Sciences and Technologies, Faculty of Sciences, Lebanese University, Fanar, Jdeidet P.O. Box 90656, Lebanon; [email protected] (H.G.-G.); [email protected] (L.A.) 3 Faculty of Medicine, LR12ES05, Lab-NAFS ‘Nutrition-Functional Food & Vascular Health’, University Monastir, Monastir 5019, Tunisia; [email protected] 4 Faculty of Medicine, University Sousse, Sousse 4000, Tunisia 5 Team OCS, Institute of Molecular Chemistry of University of Burgundy (ICMUB UMR CNRS 6302), University of Bourgogne Franche-Comté, 21000 Dijon, France; [email protected] 6 LCPMC-A2, ICPM, Depterment of Chemistry, University Lorraine, Metz Technopôle, 57070 Metz, France; [email protected] 7 Department of Physiology, School of Medicine, University College Cork, T12 Cork, Ireland; [email protected] * Correspondence: [email protected]; Tel.: +333-80-39-62-56; Fax: +333-80-39-62-50 Received: 31 July 2020; Accepted: 21 October 2020; Published: 23 October 2020 Abstract: The Mediterranean diet is associated with health benefits due to bioactive compounds such as polyphenols. -
Production of Stilbenes in Callus Cultures of the Maltese Indigenous Grapevine Variety, Gellew˙Za˙
molecules Article Production of Stilbenes in Callus Cultures of the Maltese Indigenous Grapevine Variety, Gellew˙za˙ Mariella Bonello 1, Uroš Gaši´c 2 , Živoslav Teši´c 2,* and Everaldo Attard 1 1 Division of Rural Sciences and Food Systems, Institute of Earth Systems, University of Malta, Msida MSD 2080, Malta; [email protected] (M.B.); [email protected] (E.A.) 2 University of Belgrade, Faculty of Chemistry, Studentski trg 12-16, P.O. Box 51, 11158 Belgrade, Serbia; [email protected] * Correspondence: [email protected]; Tel.: +381-113336733 Received: 14 May 2019; Accepted: 29 May 2019; Published: 4 June 2019 Abstract: The production of secondary metabolites in tissue culture has been considered as an alternative to the cultivation and harvesting of crops intended for this purpose. The present study was aimed at the growth of callus and production of polyphenolic compound of callus derived from a Maltese indigenous grapevine variety, Gellew˙za.Callus˙ was inoculated onto plant growth regulators-enriched Murashige Skoog media (MSm) to determine whether polyphenols are produced in vitro as well as to determine the best combination of plant growth regulators needed for the production of these metabolites. From results obtained, it was observed that the best callus production was obtained by auxin-enriched MSm. In fact, indole acetic acid and indole acetic acid /6-benzyl aminopurine enhanced biomass accumulation (3.04 g and 3.39 g) as opposed to the others (<1.97 g). On the other hand, parameters showing the presence of flavonoids (tonality, 3.80), particularly anthocyanins (24.09 mg/kg) and total polyphenols (1.42 mg/g), were optimum in the presence of cytokinins, particularly 6-benzyl aminopurine. -
Carotene, and Epigallocatechin Gallate (EGCG) on Amyloid-25–35 Aggregation in Synthetic Brain Membranes Wednesday, 9 June 2021 16:45 (5 Minutes)
2021 CAP Virtual Congress / Congrès virtuel de l’ACP 2021 Contribution ID: 136 Type: Oral Competition (Undergraduate Student) / Compétition orale (Étudiant(e) du 1er cycle) (U*) The Effects of Resveratrol, Caffeine, -Carotene, and Epigallocatechin Gallate (EGCG) on Amyloid-25–35 Aggregation in Synthetic Brain Membranes Wednesday, 9 June 2021 16:45 (5 minutes) Alzheimer’s disease is a neurodegenerative condition marked by the formation and aggregation of amyloid- (A) peptides. It is the most common cause of dementia worldwide, with numbers expected to double each year, reaching 81 million by 2040. There exists, to this day, no cure or effective prevention for the disease; however, there is evidence that a nutritious diet and certain food compounds can slow down first occurrence and progression of the disease. Here, we prepared synthetic membranes that contained A aggregates and investigated if certain food compounds could partition into the membrane and interact with such aggregates using optical and fluorescence microscopy, X-ray diffraction, UV–vis spectroscopy, and molecular dynamics simulations. The compounds studied were resveratrol, a polyphenol commonly found in the skin of grapes,- carotene, a naturally occurring carotenoid found in carrots, EGCG, an antioxidant abundantly found in green tea, and caffeine, the principal pharmacologically active component in coffee [1]. Evidence suggests that all compounds are membrane active and spontaneously partitioned in the membrane. While resveratrol and caffeine lead to membrane thickening and reduced membrane fluidity, -carotene and EGCG preserved or increased fluidity. Our findings show that resveratrol and caffeine did not reduce the volume fraction of peptide aggregates while -carotene significantly reduced plaque size. -
ABSTRACT SIMPSON, JOY ANNA. Antioxidant Properties of Peanut
ABSTRACT SIMPSON, JOY ANNA. Antioxidant Properties of Peanut Plant Leaves and Roots and Contribution of Specific Phenolic Compounds to Antioxidant Capacity. (Under the direction of Dr. T. H. Sanders). The peanut seeds represent less than 40 % of the total biomass of the peanut plant. Currently, peanut plants are left in the field after harvest or baled for animal feed. The research presented here was the second step in identifying bioactive compounds from peanut plants that may be the source for value added products for the peanut industry. The objective of this work was to determine the antioxidant capacity and total phenolic content for peanut leaves and roots, and to identify and quantify specific phenolic compounds contributing to the total antioxidant activity. Peanut leaves and roots were collected from a North Carolina (NC) research farm. Additional roots were collected from a Texas (TX) farm. Plant parts were freeze dried and extracted with methanol and aqueous methanol. Antioxidant activity of the extracts was tested using ORAC and DPPH assays. Total phenolics were measured using the Folin-Ciocalteau method. Chlorophyll was tested with the ORAC assay to dispel concern for potential chlorophyll antioxidant activity. Thin Layer Chromatography (TLC) and High Performance Liquid Chromatography (HPLC) were used for separation and preliminary identification of compounds contributing to the total antioxidant capacity of the peanut leaves and roots. HPLC coupled with Mass Spectrometry (MS) was utilized for identification and quantification of individual phenolic compounds in the peanut plant parts. The identified phenolic compounds were assayed individually and as a quantitative mixture with both antioxidant assays to determine the contribution of the individual compounds to the total antioxidant activity previously determined for peanut plant extracts. -
Effects of Prepubertal Exposure to Xenoestrogen on Development of Estrogen Target Organs in Female CD-1 Mice
in vivo 19: 487-494 (2005) Effects of Prepubertal Exposure to Xenoestrogen on Development of Estrogen Target Organs in Female CD-1 Mice YASUYOSHI NIKAIDO, NAOYUKI DANBARA, MIKI TSUJITA-KYUTOKU, TAKASHI YURI, NORIHISA UEHARA and AIRO TSUBURA Department of Pathology II, Kansai Medical University, Moriguchi, Osaka 570-8506, Japan Abstract. Background: There have been no previous reports more time in the estrus phase; ZEA-treated mice had a longer comparing the effects of prepubertal xenoestrogen exposure on period of anovulatory ovary than other xenoestrogen-treated development of the reproductive tract and mammary glands in mice; however, none of the xenoestrogens tested altered the female mice. The effects of genistein (GEN), resveratrol uterine or vaginal morphology or mammary gland growth. (RES), zearalenone (ZEA), zeranol (ZER), bisphenol A (BPA) and diethylstilbestrol (DES) were examined. Materials Xenoestrogens (chemicals with estrogenic activity) are and Methods: Beginning at 15 days of age, female CD-1 mice endocrine-disrupting chemicals that include naturally were administered 4 daily subcutaneous injections of 10 occurring substances produced by plants (phytoestrogens), mg/kg/day of GEN, RES, ZEA, ZER or BPA, or 10 Ìg/kg/day molds (mycoestrogens) and man-made chemicals released of DES dissolved in dimethylsulfoxide (DMSO), or DMSO into the environment (1). They may be ingested directly in vehicle. Vaginal opening was checked; estrous cyclicity was plant material, in the tissues of animals that ingest monitored from 5, 9 or 21 weeks of age for 21 consecutive xenoestrogen-producing plants or plants infected by days; 6 animals per group were autopsied at 4, 8 and 24 weeks xenoestrogen-producing molds, or in foodstuffs contaminated of age.