Schantl Et Al 2019 Combined.Pdf
Total Page:16
File Type:pdf, Size:1020Kb
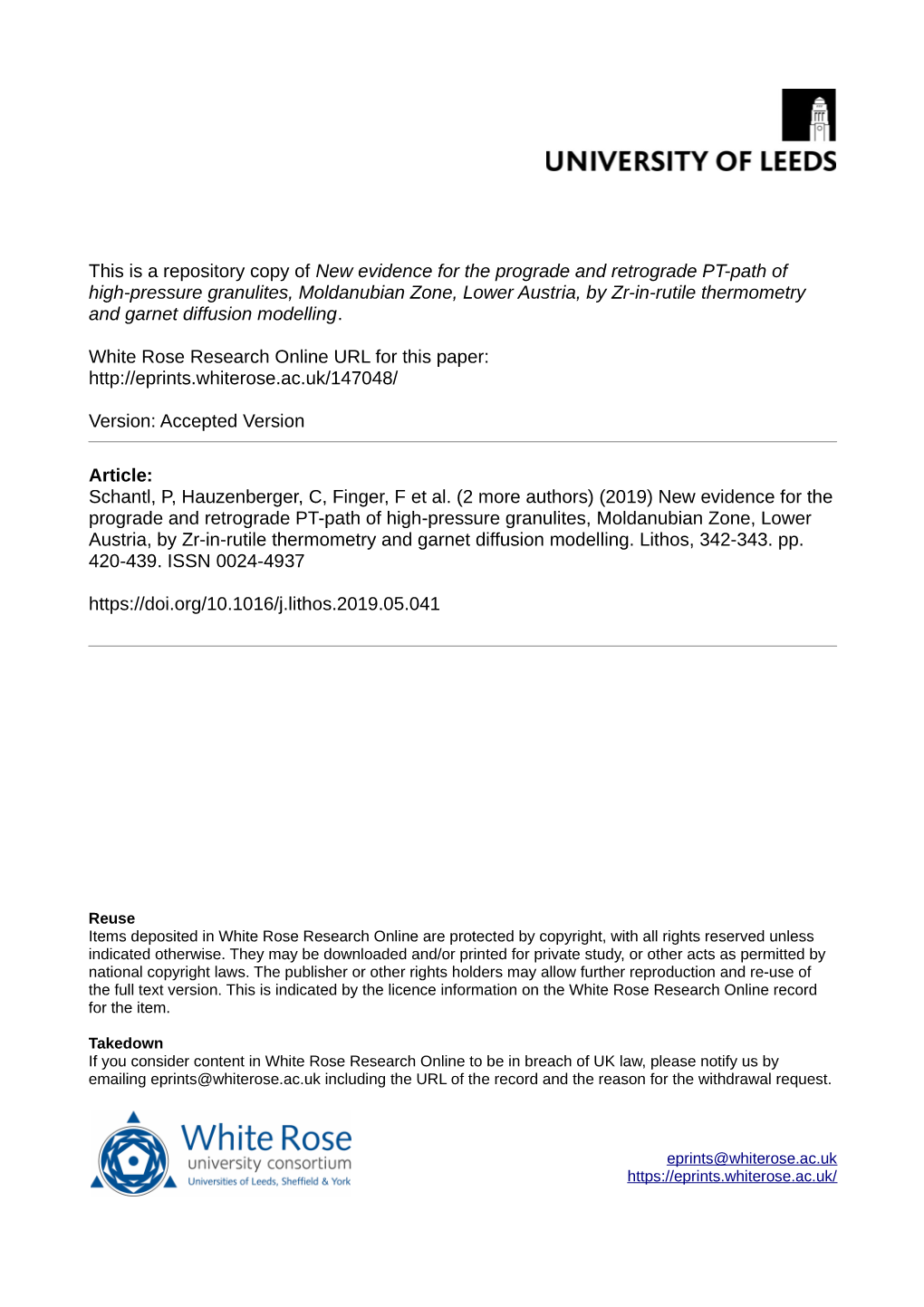
Load more
Recommended publications
-
Resolving the Variscan Evolution of the Moldanubian Sector of The
Journal of Geosciences, 52 (2007), 9–28 DOI: 10.3190/jgeosci.005 Original paper Resolving the Variscan evolution of the Moldanubian sector of the Bohemian Massif: the significance of the Bavarian and the Moravo–Moldanubian tectonometamorphic phases Fritz FINGER1*, Axel GERDEs2, Vojtěch JANOušEk3, Miloš RENé4, Gudrun RIEGlER1 1University of Salzburg, Division of Mineralogy, Hellbrunnerstraße 34, A-5020 Salzburg, Austria; [email protected] 2University of Frankfurt, Institute of Geoscience, Senckenberganlage 28, D-60054 Frankfurt, Germany 3Czech Geological Survey, Klárov 3, 118 21 Prague 1, Czech Republic 4Academy of Sciences, Institute of Rock Structure and Mechanics, V Holešovičkách 41, 182 09 Prague 8, Czech Republic *Corresponding author The Variscan evolution of the Moldanubian sector in the Bohemian Massif consists of at least two distinct tectonome- tamorphic phases: the Moravo–Moldanubian Phase (345–330 Ma) and the Bavarian Phase (330–315 Ma). The Mora- vo–Moldanubian Phase involved the overthrusting of the Moldanubian over the Moravian Zone, a process which may have followed the subduction of an intervening oceanic domain (a part of the Rheiic Ocean) beneath a Moldanubian (Armorican) active continental margin. The Moravo–Moldanubian Phase also involved the exhumation of the HP–HT rocks of the Gföhl Unit into the Moldanubian middle crust, represented by the Monotonous and Variegated series. The tectonic emplacement of the HP–HT rocks was accompanied by intrusions of distinct magnesio-potassic granitoid melts (the 335–338 Ma old Durbachite plutons), which contain components from a strongly enriched lithospheric mantle source. Two parallel belts of HP–HT rocks associated with Durbachite intrusions can be distinguished, a western one at the Teplá–Barrandian and an eastern one close to the Moravian boundary. -
The Geology of England – Critical Examples of Earth History – an Overview
The Geology of England – critical examples of Earth history – an overview Mark A. Woods*, Jonathan R. Lee British Geological Survey, Environmental Science Centre, Keyworth, Nottingham, NG12 5GG *Corresponding Author: Mark A. Woods, email: [email protected] Abstract Over the past one billion years, England has experienced a remarkable geological journey. At times it has formed part of ancient volcanic island arcs, mountain ranges and arid deserts; lain beneath deep oceans, shallow tropical seas, extensive coal swamps and vast ice sheets; been inhabited by the earliest complex life forms, dinosaurs, and finally, witnessed the evolution of humans to a level where they now utilise and change the natural environment to meet their societal and economic needs. Evidence of this journey is recorded in the landscape and the rocks and sediments beneath our feet, and this article provides an overview of these events and the themed contributions to this Special Issue of Proceedings of the Geologists’ Association, which focuses on ‘The Geology of England – critical examples of Earth History’. Rather than being a stratigraphic account of English geology, this paper and the Special Issue attempts to place the Geology of England within the broader context of key ‘shifts’ and ‘tipping points’ that have occurred during Earth History. 1. Introduction England, together with the wider British Isles, is blessed with huge diversity of geology, reflected by the variety of natural landscapes and abundant geological resources that have underpinned economic growth during and since the Industrial Revolution. Industrialisation provided a practical impetus for better understanding the nature and pattern of the geological record, reflected by the publication in 1815 of the first geological map of Britain by William Smith (Winchester, 2001), and in 1835 by the founding of a national geological survey. -
Trans-Lithospheric Diapirism Explains the Presence of Ultra-High Pressure
ARTICLE https://doi.org/10.1038/s43247-021-00122-w OPEN Trans-lithospheric diapirism explains the presence of ultra-high pressure rocks in the European Variscides ✉ Petra Maierová1 , Karel Schulmann1,2, Pavla Štípská1,2, Taras Gerya 3 & Ondrej Lexa 4 The classical concept of collisional orogens suggests that mountain belts form as a crustal wedge between the downgoing and overriding plates. However, this orogenic style is not compatible with the presence of (ultra-)high pressure crustal and mantle rocks far from the plate interface in the Bohemian Massif of Central Europe. Here we use a comparison between geological observations and thermo-mechanical numerical models to explain their formation. 1234567890():,; We suggest that continental crust was first deeply subducted, then flowed laterally under- neath the lithosphere and eventually rose in the form of large partially molten trans- lithospheric diapirs. We further show that trans-lithospheric diapirism produces a specific rock association of (ultra-)high pressure crustal and mantle rocks and ultra-potassic magmas that alternates with the less metamorphosed rocks of the upper plate. Similar rock asso- ciations have been described in other convergent zones, both modern and ancient. We speculate that trans-lithospheric diapirism could be a common process. 1 Center for Lithospheric Research, Czech Geological Survey, Prague 1, Czech Republic. 2 EOST, Institute de Physique de Globe, Université de Strasbourg, Strasbourg, France. 3 Institute of Geophysics, Department of Earth Science, ETH-Zurich, -
Pre-Mesozoic Geology of Saxo-Thuringia from the Cadomian Active Margin to the Variscan Orogen
GEOLOGICA CARPATHICA, FEBRUARY 2011, 62, 1, 42 BOOK REVIEW Pre-Mesozoic Geology of Saxo-Thuringia From the Cadomian Active Margin to the Variscan Orogen Editors: ULF LINNEMANN & ROLF L. ROMER 26–28 April 2010 2010. Schweizerbart Science Publishers, Stuttgart. 488 pages, 190 figures, 6 tables, 1 Sheet Map, 1 DVD, 1 foldout, 25x 17 cm, 1400 g. Language: English ISBN 978-3-510-65259-4, bound, price: 84.90 EUR The monographic work is a summary of results of a wider team of authors, who have contributed to the five dominant Parts, each of which consists of several Chapters focused on individual problems of the Saxo-Thuringian Region, its geological structure and its development in time and space. It is a classical analysis of the development of the Pre-Mesozoic stage from the Cadomian Active Margin to the Variscan Orogene. Saxo-Thuringia represents a very important region displaying development of the Variscides in one part of the Pangea supercontinent. The first chapter of the first part (Introduction) of the book is absolutely devoted to the location of this region of Saxo-Thuringia in the context of the Pangea supercontinent, followed by detailed recapitulation of the geological maps and results from geological mapping in historical survey from oldest documents to almost present- day works, which are well preserved and represent for the reader the classical and modern shool of regional geology of this region. The third chapter of this part completely untraditionally, but in a very interesting way shows the region of Saxo- Thuringia from the point of view of geochemical potential. -
Crystalline Complexes in the Southern Parts O.F the Bohemian Massif and in the Eastern Alps by G
INTERNATIONAL GEOLOGICAL CONGRESS XXIII SESSION PRAGUE 1968 Guide to Excursion 32 C Austria Crystalline Complexes in the Southern Parts o.f the Bohemian Massif and in the Eastern Alps by G. Frasl, H. G. Scharbert, H. Wieseneder PUBLISHED BY THE GEOLOGICAL SURVEY OF AUSTRIA Excursion 32 C / Aug. 31.-Sept. 3, 1968 Excursion Leaders and Authors of the Excursion-guide: G. FRASL Universität Salzburg, Institut für Geologie und Paläontologie - Salzburg, Porsdie straße 8. H. G. ScHARBER T Universität Wien, Mineralogisch-Petrographisches Institut - Wien I, Dr.-Karl-Lueger ring 1. H. WIESENEDER Universität Wien, Mineralogisch-Petrographisches Institut - Wien 1, Dr.-Karl-Lueger ring 1. All rights reserved. Copyright, 1968, © by Geologische Bundesanstalt, Wien III. Printed in Austria. Contents I. The Bohernian Massif in Austria, Moldanubian Zone. H. G. ScHARBERT (First day) . 5 Introduction . 5 The excursion (Stop 1/1-stop I/6) 9 II. The Bohemian Massif in Austria, Moravian Zone. G. FRASL (Second day) . 13 General remarks . 13 The excursion (Stop II/1-stop II/9) 17 III. The eastern End of the Central Alps. H. WIESENEDER (Third and fo*th day) 25 General geology of the area . 25 The Wechsel unit . 25 The "grobgneiss" formation . 26 Permo-Mesozoic . 28 Metamorphism in the Semmering-Wechsel area . 30 The excursion (Stop III/1-stop III/11) . 30 Selected literature within ead1 article 1 (First day) The Bohemian Massif in Austria The Moldanubian Zone By H. G. ScHARBERT With 1 figure Introduction The Bohemian Massif is a deeply eroded cratogenic block having re ceived its last tectonic coining during the Variscian orogeny. -
Ages of Detrital Zircons
Elsevier Editorial System(tm) for Precambrian Research Manuscript Draft Manuscript Number: Title: AGES OF DETRITAL ZIRCONS (U/Pb, LA-ICP-MS) FROM THE LATEST NEOPROTEROZOIC - MIDDLE CAMBRIAN(?) ASHA GROUP AND EARLY DEVONIAN TAKATY FORMATION, THE SOUTH- WESTERN URALS: A TEST OF AN AUSTRALIA-BALTICA CONNECTION WITHIN RODINIA Article Type: SI:Precambrian Supercontinents Keywords: Urals, Detrital Zircon, Rodinia, Ediacaran, Baltica Corresponding Author: Prof. Nikolay Borisovich Kuznetsov, Ph.D. Corresponding Author's Institution: Geological Institute of Russian Academy of Science First Author: Nikolay B Kuznetsov, Ph.D. Order of Authors: Nikolay B Kuznetsov, Ph.D.; Josef G Meert, Ph.D.; Tatiana V Romanyuk, Ph.D. Abstract: Results from U/Pb-dating of detrital zircons (dZr) from sandstones of the Basu and Kukkarauk Fms. (Asha Group) of Ediacaran-Middle Cambrian(?) age along with the results obtained from the Early Devonian Takaty Fm. are presented. The age of the Asha Group is traditionally labeled as Upper Vendian in the Russian stratigraphic chart that overlaps with the Ediacaran in the International stratigraphic chart. The dZr whose ages fall within the age-interval of (500-750 Ma) are common in the Basu and Kukkarauk Fm. These ages are typical for crystalline complexes in the Pre- Uralides-Timanides orogen. The identification of zircons with this age range agrees with commonly adopted interpretations for the depositional origin of the Asha Group as a molasse resulting from the erosion of that orogenic belt. Based on the estimates of the youngest ages of dZr along with the tentative identification of inarticulate brachiopods in the Kukkarauk Fm., it appears that the upper part of the Asha Group may extend into the Middle Cambrian. -
Lower Palaeozoic Evolution of the Northeast German Basin/Baltica Borderland
Originally published as: McCann, T. (1998): Lower Palaeozoic evolution of the NE German Basin/Baltica borderland. - Geological Magazine, 135, 129-142. DOI: 10.1017/S0016756897007863 Geol. Mag. 135 (1), 1998, pp. 129–142. Printed in the United Kingdom © 1998 Cambridge University Press 129 Lower Palaeozoic evolution of the northeast German Basin/Baltica borderland TOMMY MCCANN GeoForschungsZentrum (Projektbereich 3.3 – Sedimente und Beckenbildung), Telegrafenberg A26, 14473 Potsdam, Germany (Received 15 October 1996; accepted 11 July 1997) Abstract – The Vendian–Silurian succession from a series of boreholes in northeast Germany has been pet- rographically and geochemically investigated. Evidence suggests that the more northerly Vendian and Cambrian succession was deposited on a craton which became increasingly unstable in Ordovician times. Similarly, the Ordovician-age succession deposited in the Rügen area indicates a strongly active continental margin tectonic setting for the same period. By Silurian times the region was once more relatively tectoni- cally quiescent. Although complete closure of the Tornquist Sea was not complete until latest Silurian times, the major changes in tectonic regime in the Eastern Avalonia/Baltica area recorded from the Ordovician sug- gest that a significant degree of closure occurred during this time. The precise location of the southwestern edge of 1. Introduction Baltica (that is, that part of Baltica to the south of the The northeast German Basin is situated between the sta- Sorgenfrei-Tornquist Zone) is not known. This is largely ble Precambrian shield area of the Baltic Sea/Scandinavia as a result of masking by younger sediments (Tanner & to the north and the Cadomian/Caledonian/Variscan- Meissner, 1996). -
NATIONAL Fafohrabilitt STUDIES UNITED KIBGDOM 77-9974
A International Atomic Energy Agency IDREP N.F.S, Uo. 108 October 1977 Distr. LIMITS) Originals 5RENCH IHTERIATIOEAL URAHIUM 1ES0URCES EVALUATION PROJECT IUEEP NATIONAL FAfOHRABILITT STUDIES UNITED KIBGDOM 77-9974 INTERNATIONAL URAHIUM RESOURCES EVALUATION PROJECT IUEEP c NATIOHAL PAVOURABILITY STUDIES IUREP H.P.S. Bo. 108 UNITED KINGDOM CONTENTS SUMMARY PAGE A. INTRODUCTION AND GENERAL GEOGRAPHY 1. B. GEOLOGY OP THE UNITED KINGDOM IN RELATION TO POTENTIALLY FAVOURABLE URANIUM BEARING AREAS C. PAST EXPLORATION 5. D. URANIUM OCCURRENCES AND RESOURCES 6. E. PRESENT STATUS OP EXPLORATION 9 P. POTENTIAL FOR NEW DISCOVERIES 9 BIBLIOGRAPHY 13. FIGURES Bo, 1 Map of the United 'Kingdom 77-9169 Translated from French UNITED KINGDOM INTERNATIONAL URANIUM RESOURCES EVALUATION PROJECT (lUREP) SOMKARY Although uranium prospecting was commenced in the United Kingdom (area 244 8l3 km ) at the end of the last century and was resumed just after the Second World War, it does not seem, for various reasons, despite the level of competence of its specialists and the level of instrumentation available, that the country has "been adequately prospected for uranium* The small reserves discovered to date, some 7400t U for all the official NEA/lAEA categories, probably do not reflect the true uranium potential of the United Kingdom. However, they do indicate without doubt that the resources remaining to be discovered are so located that detection will be difficult. The most promising areas of investigation in our opinion are the Old Red Sandstones of the Devonian period on the one hand and the districts where the uraniferous black shales of the Cambro-Ordovician and Namurian have suffered perturbations which may have led to immobili- zation of their uranium content (in particular, granitizations)« All the considerations put forward in this analysis lead us to place the United Kingdom in category 4 of the classification adopted for IUHEP. -
Diplomová Práce
MASARYKOVA UNIVERZITA PŘÍRODOVĚDECKÁ FAKULTA ÚSTAV GEOLOGICKÝCH VĚD Diplomová práce Brno 2015 Hana Kupská MASARYKOVA UNIVERZITA PŘÍRODOVĚDECKÁ FAKULTA ÚSTAV GEOLOGICKÝCH VĚD STUDIUM DUTINOVÝCH PEGMATITŮ ČESKOMORAVSKÉ VRCHOVINY NA PŘÍKLADU PEGMATITU U STRÁŽKU, STRÁŽECKÉ MOLDANUBIKUM Diplomová práce Hana Kupská Vedoucí práce: Mgr. Petr Gadas, Ph.D. Brno 2015 Bibliografický záznam Autor: Hana Kupská, Bc. Přírodovědecká fakulta, Masarykova univerzita Ústav geologických věd Název práce: Studium dutinových pegmatitů Českomoravské vrchoviny na příkladu pegmatitu u Strážku, strážecké moldanubikum Studijní program: Geologie Studijní obor: Geologie Vedoucí práce: Mgr. Petr Gadas, Ph.D. Akademický rok: 2014/2015 Počet stran: 55 Klíčová slova: Granitický pegmatit; dutinový pegmatit; živce; slídy; turmalíny; granáty; optická mikroskopie; elektronová mikrosonda; strážecké moldanubikum; Českomoravská vrchovina; Strážek Bibliographic entry Author: Hana Kupská, Bc. Faculty of Science, Masaryk University Department of geology Title of thesis: Study of pegmatites with pockets from the Českomoravská Highland - case study of pegmatite from Strážek, Strážek Moldanubicum Degree programme: Geology Field of study: Geology Supervisor: Mgr. Petr Gadas, Ph.D. Academic year: 2014/2015 Number of pages: 55 Keywords: Granitic pegmatite; pocket pegmatite; feldspars; micas; tourmalines; garnets; optical microscopy; electron microprobe; Strážek Moldanubicum; Českomoravská Highland; Strážek Abstrakt Tato diplomová práce se zabývá mineralogií a petrologií dutinového pegmatitu -
Tectonic Evolution of the Mesozoic South Anyui Suture Zone, GEOSPHERE; V
Research Paper GEOSPHERE Tectonic evolution of the Mesozoic South Anyui suture zone, GEOSPHERE; v. 11, no. 5 eastern Russia: A critical component of paleogeographic doi:10.1130/GES01165.1 reconstructions of the Arctic region 18 figures; 5 tables; 2 supplemental files; 1 animation Jeffrey M. Amato1, Jaime Toro2, Vyacheslav V. Akinin3, Brian A. Hampton1, Alexander S. Salnikov4, and Marianna I. Tuchkova5 1Department of Geological Sciences, New Mexico State University, MSC 3AB, P.O. Box 30001, Las Cruces, New Mexico 88003, USA CORRESPONDENCE: [email protected] 2Department of Geology and Geography, West Virginia University, 330 Brooks Hall, P.O. Box 6300, Morgantown, West Virginia 26506, USA 3North-East Interdisciplinary Scientific Research Institute, Far East Branch, Russian Academy of Sciences, Magadan, Portovaya Street, 16, 685000, Russia CITATION: Amato, J.M., Toro, J., Akinin, V.V., Hamp- 4Siberian Research Institute of Geology, Geophysics, and Mineral Resources, 67 Krasny Prospekt, Novosibirsk, 630091, Russia ton, B.A., Salnikov, A.S., and Tuchkova, M.I., 2015, 5Geological Institute, Russian Academy of Sciences, Pyzhevskii per. 7, Moscow, 119017, Russia Tectonic evolution of the Mesozoic South Anyui su- ture zone, eastern Russia: A critical component of paleogeographic reconstructions of the Arctic region: ABSTRACT INTRODUCTION Geosphere, v. 11 no. 5, p. 1530–1564, doi: 10 .1130 /GES01165.1. The South Anyui suture zone consists of late Paleozoic–Jurassic ultra- The South Anyui suture zone (Fig. 1) is a remnant of a Mesozoic ocean Received 19 December 2014 mafic rocks and Jurassic–Cretaceous pre-, syn-, and postcollisional sedimen- basin that separated the Arctic Alaska–Chukotka microplate from Siberia Revision received 2 July 2015 tary rocks. -
Paleozoic Evolution of Pre-Variscan Terranes: from Gondwana to the Variscan Collision
Geological Society of America Special Paper 364 2002 Paleozoic evolution of pre-Variscan terranes: From Gondwana to the Variscan collision Gérard M. Stamp×i Institut de Géologie et Paléontologie, Université de Lausanne, CH-1015 Lausanne, Switzerland Jürgen F. von Raumer Institut de Minéralogie et Pétrographie, Université de Fribourg, CH-1700 Fribourg, Switzerland Gilles D. Borel Institut de Géologie et Paléontologie, Université de Lausanne, CH-1015 Lausanne, Switzerland ABSTRACT The well-known Variscan basement areas of Europe contain relic terranes with a pre-Variscan evolution testifying to their peri-Gondwanan origin (e.g., relics of Neo- proterozoic volcanic arcs, and subsequent stages of accretionary wedges, backarc rift- ing, and spreading). The evolution of these terranes was guided by the diachronous subduction of the proto-Tethys oceanic ridge under different segments of the Gond- wana margin. This subduction triggered the emplacement of magmatic bodies and the formation of backarc rifts, some of which became major oceanic realms (Rheic, paleo- Tethys). Consequently, the drifting of Avalonia was followed, after the Silurian and a short Ordovician orogenic event, by the drifting of Armorica and Alpine domains, ac- companied by the opening of the paleo-Tethys. The slab rollback of the Rheic ocean is viewed as the major mechanism for the drifting of the European Variscan terranes. This, in turn, generated a large slab pull force responsible for the opening of major rift zones within the passive Eurasian margin. Therefore, the µrst Middle Devonian Variscan orogenic event is viewed as the result of a collision between terranes detached from Gondwana (grouped as the Hun superterrane) and terranes detached from Eurasia. -
The Apennines, the Dinarides, and the Adriatic Sea: Is the Adriatic Microplate a Reality?
Geogr. Fis. Dinam. Quat. 32 (2009), 167-175, 13 figg. CLIFF D. OLLIER (*) & COLIN F. PAIN (**) THE APENNINES, THE DINARIDES, AND THE ADRIATIC SEA: IS THE ADRIATIC MICROPLATE A REALITY? ABSTRACT: OLLIER C.D. & PAIN C.F., The Apennines, the Dinarides, here called the Dinaride Mountains (which is sometimes and the Adriatic Sea: is the Adriatic Microplate a reality?. (IT ISSN 0391- split into different ranges in different countries, such as the 9838, 2009). Albanides in Albania) as shown in fig. 1. Structurally both The Apennines and the Dinarides consist of nappes thrust towards the Apennines and the Dinarides are thrust towards the the Adriatic Sea, which is underlain by largely undisturbed rocks. Plate tectonic reconstructions are very varied, with supposed subduction in Adriatic. The tectonic position of this area is problematic. many different directions. Besides this there is an over-ruling concept In plate tectonic terms the Dinaride Mountains are that a plate called the Adriatic (or Adria) Plate moved north from Africa usually explained as a result of subduction of a plate under to Europe where its collision helped to create the Alps. Some think the plate is still moving. The total tectonic setting, together with palaeonto- the Dinarides. Similarly the Apennines are commonly ex- logical and seismic data, suggests that the older model of two converging nappe belts meeting a common foreland best fits the observed facts. KEY WORDS: Adriatic, Apennines, Dinarides, Plates, Arcs. RIASSUNTO: OLLIER C.D. & PAIN C.F., Gli Appennini, le Dinaridi e il mare Adriatico: la Microplacca Adriatica è una realtà?. (IT ISSN 0391- 9838, 2009).