New Evidence of Meteoritic Origin of the Tunguska Cosmic Body Victor
Total Page:16
File Type:pdf, Size:1020Kb
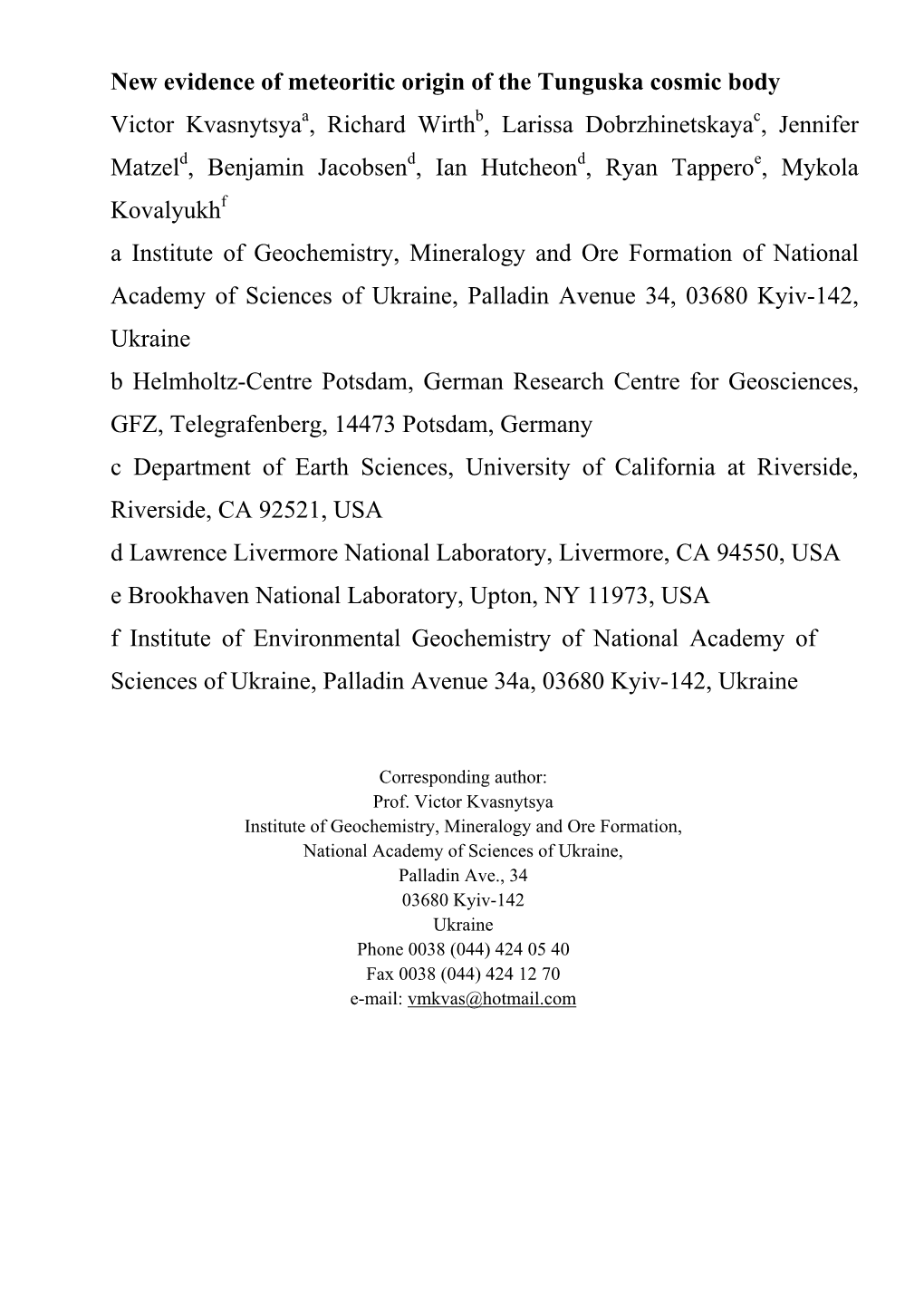
Load more
Recommended publications
-
Spring 1998 Gems & Gemology
VOLUME 34 NO. 1 SPRING 1998 TABLE OF CONTENTS EDITORIAL 1 The Dr. Edward J. Gübelin Most Valuable Article Award FEATURE ARTICLE 4 The Rise to pProminence of the Modern Diamond Cutting Industry in India Menahem Sevdermish, Alan R. Miciak, and Alfred A. Levinson pg. 7 NOTES AND NEW TECHNIQUES 24 Leigha: The Creation of a Three-Dimensional Intarsia Sculpture Arthur Lee Anderson 34 Russian Synthetic Pink Quartz Vladimir S. Balitsky, Irina B. Makhina, Vadim I. Prygov, Anatolii A. Mar’in, Alexandr G. Emel’chenko, Emmanuel Fritsch, Shane F. McClure, Lu Taijing, Dino DeGhionno, John I. Koivula, and James E. Shigley REGULAR FEATURES pg. 30 44 Gem Trade Lab Notes 50 Gem News 64 Gems & Gemology Challenge 66 Book Reviews 68 Gemological Abstracts ABOUT THE COVER: Over the past 30 years, India has emerged as the dominant sup- plier of small cut diamonds for the world market. Today, nearly 70% by weight of the diamonds polished worldwide come from India. The feature article in this issue discuss- es India’s near-monopoly of the cut diamond industry, and reviews India’s impact on the worldwide diamond trade. The availability of an enormous amount of small, low-cost pg. 42 Indian diamonds has recently spawned a growing jewelry manufacturing sector in India. However, the Indian diamond jewelery–making tradition has been around much longer, pg. 46 as shown by the 19th century necklace (39.0 cm long), pendant (4.5 cm high), and bracelet (17.5 cm long) on the cover. The necklace contains 31 table-cut diamond panels, with enamels and freshwater pearls. -
Experimental and Petrological Constraints on Lunar Differentiation from the Apollo 15 Green Picritic Glasses
Meteoritics & Planetary Science 38, Nr 4, 515–527(2003) Abstract available online at http://meteoritics.org Experimental and petrological constraints on lunar differentiation from the Apollo 15 green picritic glasses Linda T. ELKINS-TANTON,1* Nilanjan CHATTERJEE,2 and Timothy L. GROVE2 1Department of Geological Sciences, Brown University, Providence, Rhode Island, 02912, USA 2Department of Earth, Atmospheric and Planetary Sciences, Massachusetts Institute of Technology, Cambridge, Massachusetts 02139, USA *Corresponding author: [email protected] (Received 15 July 2002; revision accepted 23 September 2002) Abstract–Phase equilibrium experiments on the most magnesian Apollo 15C green picritic glass composition indicate a multiple saturation point with olivine and orthopyroxene at 1520°C and 1.3 GPa (about 260 km depth in the moon). This composition has the highest Mg# of any lunar picritic glass and the shallowest multiple saturation point. Experiments on an Apollo 15A composition indicate a multiple saturation point with olivine and orthopyroxene at 1520°C and 2.2 GPa (about 440 km depth in the moon). The importance of the distinctive compositional trends of the Apollo 15 groups A, B, and C picritic glasses merits the reanalysis of NASA slide 15426,72 with modern electron microprobe techniques. We confirm the compositional trends reported by Delano (1979, 1986) in the major element oxides SiO2, TiO2, Al2O3, Cr2O3, FeO, MnO, MgO, and CaO, and we also obtained data for the trace elements P2O5, K2O, Na2O, NiO, S, Cu, Cl, Zn, and F. Petrogenetic modeling demonstrates that the Apollo 15 A-B-C glass trends could not have been formed by fractional crystallization or any continuous assimilation/fractional crystallization (AFC) process. -
Evolution of the Iron–Silicate and Carbon Material of Carbonaceous Chondrites A
ISSN 01458752, Moscow University Geology Bulletin, 2013, Vol. 68, No. 5, pp. 265–281. © Allerton Press, Inc., 2013. Original Russian Text © A.A. Marakushev, L.I. Glazovskaya, S.A. Marakushev, 2013, published in Vestnik Moskovskogo Universiteta. Geologiya, 2013, No. 5, pp. 3–17. Evolution of the Iron–Silicate and Carbon Material of Carbonaceous Chondrites A. A. Marakusheva, L. I. Glazovskayab, and S. A. Marakushevc aInstitute of Experimental Mineralogy, Russian Academy of Sciences, Chernogolovka, Moscow oblast, 142432 Russia email: [email protected] bFaculty of Geology, Moscow State University, Moscow, 119234 Russia email: [email protected] cInstitute of Problems of Chemical Physics, Russian Academy of Sciences, Chernogolovka, Moscow oblast, 142432 Russia email: [email protected] Received February 18, 2013 Abstract—The observed consistence of the composition of chondrules and the matrix in chondrites is explained by their origin as a result of chondrule–matrix splitting of the material of primitive (not layered) planets. According to the composition of chondrites, two main stages in the evolution of chondritic planets (silicate–metallic and olivine) are distinguished. Chondritic planets of the silicate–metallic stage were ana logs of chondritic planets, whose layering resulted in the formation of the terrestrial planets. The iron–silicate evolution of chondritic matter is correlated with the evolution of carbon material in the following sequence: diamond ± moissanite → hydrocarbons → primitive organic compounds. Keywords: meteorite matter, carbonaceous chondrites, hydrocarbons DOI: 10.3103/S0145875213050074 INTRODUCTION 2003) in star–planet systems that are similar to the solar system, in which nearstar giant planets were still The occurrence of diamond in a stable paragenesis preserved and are distinguished under the name rapid with moissanite (SiC) in an ironrich matrix is a char Jupiters. -
Lunar and Planetary Science XXX 1753.Pdf
Lunar and Planetary Science XXX 1753.pdf INTERSTELLAR DIAMOND. II. GROWTH AND IDENTIFICATION. Andrew W. Phelps, University of Dayton Research Institute, Materials Engineering Division (300 College Park, Dayton OH, 45469-0130, [email protected]). likely form clumps of carbon that look much like the Introduction: The first abstract in this series starting material – hydrogen rich sp3 bound clusters. suggested that many types of diamond are found in many types of meteoritic material and that the The compounds that fit the requirements above variation in polytype abundance appears to reflect the include the cycloalkanes such as adamantane [21-30] nature of the host. How the diamonds got there and (C10H16) and bicyclooctane (C6H8) (BCO) . what they mean will now be examined. Opinions These compounds ‘look’ like small cubic diamonds regarding the formation mechanism(s) of meteoritic and lonsdaleite respectively. If these particular and interstellar diamond grains have changed over compounds are suggested as models for diamond the years as new methods of making synthetic nuclei then a number of structural and diamond were developed[1,2]. Meteoritic diamonds thermodynamic predictions can be made about the were once believed to be the result of high pressure - types of diamond that can form and its relative temperature processes in the interior of planetary abundance with regard to the physics and chemistry bodies[3,4]. Impact formation of diamond became of its growth environment. A few examples of this popular after the development of shock wave are: Adamantane is a lower energy form and would diamond synthesis[5] because shock synthesis was tend to form in an environment of sufficient thermal able to form lonsdaleite and meteorites were full of energy to allow the molecule to organize into this lonsdaleite. -
Impact−Cosmic−Metasomatic Origin of Microdiamonds from Kumdy−Kol Deposit, Kokchetav Massiv, N
11th International Kimberlite Conference Extended Abstract No. 11IKC-4506, 2017 Impact−Cosmic−Metasomatic Origin of Microdiamonds from Kumdy−Kol Deposit, Kokchetav Massiv, N. Kazakhstan L. I. Tretiakova1 and A. M. Lyukhin2 1St. Petersburg Branch Russian Mineralogical Society. RUSSIA, [email protected] 2Institute of remote ore prognosis, Moscow, RUSSIA, [email protected] Introduction Any collision extraterrestrial body and the Earth had left behind the “signature” on the Earth’s surface. We are examining a lot of signatures of an event caused Kumdy-Kol diamond-bearing deposit formation, best- known as “metamorphic” diamond locality among numerous UHP terrains around the world. We are offering new impact-cosmic-metasomatic genesis of this deposit and diamond origin provoked by impact event followed prograde and retrograde metamorphism with metasomatic alterations of collision area rocks that have been caused of diamond nucleation, growth and preserve. Brief geology of Kumdy-Kol diamond-bearing deposit Kumdy-Kol diamond-bearing deposit located within ring structure ~ 4 km diameter, in the form and size compares with small impact crater (Fig. 1). It is important impact event signature. Figure1: Cosmic image of Kumdy-Kol deposit area [http://map.google.ru/]. Diamond-bearing domain had been formed on the peak of UHP metamorphism provoked by comet impact under oblique angle on the Earth surface. As a result, steep falling system of tectonic dislocations, which breakage and fracture zones filling out of impact and host rock breccia with blastomylonitic and blastocataclastic textures have been created. Diamond-bearing domain has complicated lenticular-bloc structure (1300 x 40-200 m size) and lens out with deep about 300 m. -
Chondrule Sizes, We Have Compiled and Provide Commentary on Available Chondrule Dimension Literature Data
Invited review Chondrule size and related physical properties: a compilation and evaluation of current data across all meteorite groups. Jon M. Friedricha,b,*, Michael K. Weisbergb,c,d, Denton S. Ebelb,d,e, Alison E. Biltzf, Bernadette M. Corbettf, Ivan V. Iotzovf, Wajiha S. Khanf, Matthew D. Wolmanf a Department of Chemistry, Fordham University, Bronx, NY 10458 USA b Department of Earth and Planetary Sciences, American Museum of Natural History, New York, NY 10024 USA c Department of Physical Sciences, Kingsborough College of the City University of New York, Brooklyn, NY 11235, USA d Graduate Center of the City University of New York, 365 5th Ave, New York, NY 10016 USA e Lamont-Doherty Earth Observatory, Columbia University, Palisades, New York 10964 USA f Fordham College at Rose Hill, Fordham University, Bronx, NY 10458 USA In press in Chemie der Erde – Geochemistry 21 August 2014 *Corresponding Author. Tel: +718 817 4446; fax: +718 817 4432. E-mail address: [email protected] 2 ABSTRACT The examination of the physical properties of chondrules has generally received less emphasis than other properties of meteorites such as their mineralogy, petrology, and chemical and isotopic compositions. Among the various physical properties of chondrules, chondrule size is especially important for the classification of chondrites into chemical groups, since each chemical group possesses a distinct size-frequency distribution of chondrules. Knowledge of the physical properties of chondrules is also vital for the development of astrophysical models for chondrule formation, and for understanding how to utilize asteroidal resources in space exploration. To examine our current knowledge of chondrule sizes, we have compiled and provide commentary on available chondrule dimension literature data. -
Handbook of Iron Meteorites, Volume 2 (Canyon Diablo, Part 2)
Canyon Diablo 395 The primary structure is as before. However, the kamacite has been briefly reheated above 600° C and has recrystallized throughout the sample. The new grains are unequilibrated, serrated and have hardnesses of 145-210. The previous Neumann bands are still plainly visible , and so are the old subboundaries because the original precipitates delineate their locations. The schreibersite and cohenite crystals are still monocrystalline, and there are no reaction rims around them. The troilite is micromelted , usually to a somewhat larger extent than is present in I-III. Severe shear zones, 100-200 J1 wide , cross the entire specimens. They are wavy, fan out, coalesce again , and may displace taenite, plessite and minerals several millimeters. The present exterior surfaces of the slugs and wedge-shaped masses have no doubt been produced in a similar fashion by shear-rupture and have later become corroded. Figure 469. Canyon Diablo (Copenhagen no. 18463). Shock The taenite rims and lamellae are dirty-brownish, with annealed stage VI . Typical matte structure, with some co henite crystals to the right. Etched. Scale bar 2 mm. low hardnesses, 160-200, due to annealing. In crossed Nicols the taenite displays an unusual sheen from many small crystals, each 5-10 J1 across. This kind of material is believed to represent shock annealed fragments of the impacting main body. Since the fragments have not had a very long flight through the atmosphere, well developed fusion crusts and heat-affected rim zones are not expected to be present. The energy responsible for bulk reheating of the small masses to about 600° C is believed to have come from the conversion of kinetic to heat energy during the impact and fragmentation. -
Lifetimes of Interstellar Dust from Cosmic Ray Exposure Ages of Presolar Silicon Carbide
Lifetimes of interstellar dust from cosmic ray exposure ages of presolar silicon carbide Philipp R. Hecka,b,c,1, Jennika Greera,b,c, Levke Kööpa,b,c, Reto Trappitschd, Frank Gyngarde,f, Henner Busemanng, Colin Madeng, Janaína N. Ávilah, Andrew M. Davisa,b,c,i, and Rainer Wielerg aRobert A. Pritzker Center for Meteoritics and Polar Studies, The Field Museum of Natural History, Chicago, IL 60605; bChicago Center for Cosmochemistry, The University of Chicago, Chicago, IL 60637; cDepartment of the Geophysical Sciences, The University of Chicago, Chicago, IL 60637; dNuclear and Chemical Sciences Division, Lawrence Livermore National Laboratory, Livermore, CA 94550; ePhysics Department, Washington University, St. Louis, MO 63130; fCenter for NanoImaging, Harvard Medical School, Cambridge, MA 02139; gInstitute of Geochemistry and Petrology, ETH Zürich, 8092 Zürich, Switzerland; hResearch School of Earth Sciences, The Australian National University, Canberra, ACT 2601, Australia; and iEnrico Fermi Institute, The University of Chicago, Chicago, IL 60637 Edited by Mark H. Thiemens, University of California San Diego, La Jolla, CA, and approved December 17, 2019 (received for review March 15, 2019) We determined interstellar cosmic ray exposure ages of 40 large ago. These grains are identified as presolar by their large isotopic presolar silicon carbide grains extracted from the Murchison CM2 anomalies that exclude an origin in the Solar System (13, 14). meteorite. Our ages, based on cosmogenic Ne-21, range from 3.9 ± Presolar stardust grains are the oldest known solid samples 1.6 Ma to ∼3 ± 2 Ga before the start of the Solar System ∼4.6 Ga available for study in the laboratory, represent the small fraction ago. -
Evidence from Polymict Ureilite Meteorites for a Disrupted and Re-Accreted Single Ureilite Parent Asteroid Gardened by Several Distinct Impactors
Available online at www.sciencedirect.com Geochimica et Cosmochimica Acta 72 (2008) 4825–4844 www.elsevier.com/locate/gca Evidence from polymict ureilite meteorites for a disrupted and re-accreted single ureilite parent asteroid gardened by several distinct impactors Hilary Downes a,b,*, David W. Mittlefehldt c, Noriko T. Kita d, John W. Valley d a School of Earth Sciences, Birkbeck University of London, Malet Street, London WC1E 7HX, UK b Lunar and Planetary Institute, 3600 Bay Area Boulevard, Houston, TX 77058, USA c Mail Code KR, NASA/Johnson Space Center, Houston, TX 77058, USA d Department of Geology and Geophysics, University of Wisconsin-Madison, 1215 W. Dayton St., Madison, WI 53706, USA Received 25 July 2007; accepted in revised form 24 June 2008; available online 17 July 2008 Abstract Ureilites are ultramafic achondrites that exhibit heterogeneity in mg# and oxygen isotope ratios between different meteor- ites. Polymict ureilites represent near-surface material of the ureilite parent asteroid(s). Electron microprobe analyses of >500 olivine and pyroxene clasts in several polymict ureilites reveal a statistically identical range of compositions to that shown by unbrecciated ureilites, suggesting derivation from a single parent asteroid. Many ureilitic clasts have identical compositions to the anomalously high Mn/Mg olivines and pyroxenes from the Hughes 009 unbrecciated ureilite (here termed the ‘‘Hughes cluster”). Some polymict samples also contain lithic clasts derived from oxidized impactors. The presence of several common distinctive lithologies within polymict ureilites is additional evidence that ureilites were derived from a single parent asteroid. In situ oxygen three isotope analyses were made on individual ureilite minerals and lithic clasts, using a secondary ion mass spectrometer (SIMS) with precision typically better than 0.2–0.4& (2SD) for d18O and d17O. -
Addibischoffite, Ca2al6al6o20, a New Calcium Aluminate Mineral from The
1 Revision 3 2 Addibischoffite, Ca2Al6Al6O20, a new calcium aluminate mineral from 3 the Acfer 214 CH carbonaceous chondrite: A new refractory phase from 4 the solar nebula 5 Chi Ma1,*, Alexander N. Krot2, Kazuhide Nagashima2 6 1Division of Geological and Planetary Sciences, California Institute of Technology, 7 Pasadena, California 91125, USA 8 2Hawai‘i Institute of Geophysics and Planetology, University of Hawai‘i at Mānoa, 9 Honolulu, Hawai‘i 96822, USA 10 11 ABSTRACT 12 Addibischoffite (IMA 2015-006), Ca2Al6Al6O20, is a new calcium aluminate mineral 13 that occurs with hibonite, perovskite, kushiroite, Ti-kushiroite, spinel, melilite, 14 anorthite and FeNi-metal in the core of a Ca-Al-rich inclusion (CAI) in the Acfer 15 214 CH3 carbonaceous chondrite. The mean chemical composition of type 16 addibischoffite by electron probe microanalysis is (wt%) Al2O3 44.63, CaO 15.36, 17 SiO2 14.62, V2O3 10.64, MgO 9.13, Ti2O3 4.70, FeO 0.46, total 99.55, giving rise to 18 an empirical formula of 3+ 3+ 2+ 19 (Ca2.00)(Al2.55Mg1.73V 1.08Ti 0.50Ca0.09Fe 0.05)Σ6.01(Al4.14Si1.86)O20. The general 20 formula is Ca2(Al,Mg,V,Ti)6(Al,Si)6O20. The end-member formula is Ca2Al6Al6O20. 21 Addibischoffite has the P1 aenigmatite structure with a = 10.367 Å, b = 10.756 Å, c 22 = 8.895 Å, α = 106.0°, β = 96.0°, γ = 124.7°, V = 739.7 Å3, and Z = 2, as revealed by 23 electron back-scatter diffraction. The calculated density using the measured 24 composition is 3.41 g/cm3. -
POSTER SESSION 5:30 P.M
Monday, July 27, 1998 POSTER SESSION 5:30 p.m. Edmund Burke Theatre Concourse MARTIAN AND SNC METEORITES Head J. W. III Smith D. Zuber M. MOLA Science Team Mars: Assessing Evidence for an Ancient Northern Ocean with MOLA Data Varela M. E. Clocchiatti R. Kurat G. Massare D. Glass-bearing Inclusions in Chassigny Olivine: Heating Experiments Suggest Nonigneous Origin Boctor N. Z. Fei Y. Bertka C. M. Alexander C. M. O’D. Hauri E. Shock Metamorphic Features in Lithologies A, B, and C of Martian Meteorite Elephant Moraine 79001 Flynn G. J. Keller L. P. Jacobsen C. Wirick S. Carbon in Allan Hills 84001 Carbonate and Rims Terho M. Magnetic Properties and Paleointensity Studies of Two SNC Meteorites Britt D. T. Geological Results of the Mars Pathfinder Mission Wright I. P. Grady M. M. Pillinger C. T. Further Carbon-Isotopic Measurements of Carbonates in Allan Hills 84001 Burckle L. H. Delaney J. S. Microfossils in Chondritic Meteorites from Antarctica? Stay Tuned CHONDRULES Srinivasan G. Bischoff A. Magnesium-Aluminum Study of Hibonites Within a Chondrulelike Object from Sharps (H3) Mikouchi T. Fujita K. Miyamoto M. Preferred-oriented Olivines in a Porphyritic Olivine Chondrule from the Semarkona (LL3.0) Chondrite Tachibana S. Tsuchiyama A. Measurements of Evaporation Rates of Sulfur from Iron-Iron Sulfide Melt Maruyama S. Yurimoto H. Sueno S. Spinel-bearing Chondrules in the Allende Meteorite Semenenko V. P. Perron C. Girich A. L. Carbon-rich Fine-grained Clasts in the Krymka (LL3) Chondrite Bukovanská M. Nemec I. Šolc M. Study of Some Achondrites and Chondrites by Fourier Transform Infrared Microspectroscopy and Diffuse Reflectance Spectroscopy Semenenko V. -
Impact Shock Origin of Diamonds in Ureilite Meteorites
Impact shock origin of diamonds in ureilite meteorites Fabrizio Nestolaa,b,1, Cyrena A. Goodrichc,1, Marta Moranad, Anna Barbarod, Ryan S. Jakubeke, Oliver Christa, Frank E. Brenkerb, M. Chiara Domeneghettid, M. Chiara Dalconia, Matteo Alvarod, Anna M. Fiorettif, Konstantin D. Litasovg, Marc D. Friesh, Matteo Leonii,j, Nicola P. M. Casatik, Peter Jenniskensl, and Muawia H. Shaddadm aDepartment of Geosciences, University of Padova, I-35131 Padova, Italy; bGeoscience Institute, Goethe University Frankfurt, 60323 Frankfurt, Germany; cLunar and Planetary Institute, Universities Space Research Association, Houston, TX 77058; dDepartment of Earth and Environmental Sciences, University of Pavia, I-27100 Pavia, Italy; eAstromaterials Research and Exploration Science Division, Jacobs Johnson Space Center Engineering, Technology and Science, NASA, Houston, TX 77058; fInstitute of Geosciences and Earth Resources, National Research Council, I-35131 Padova, Italy; gVereshchagin Institute for High Pressure Physics RAS, Troitsk, 108840 Moscow, Russia; hNASA Astromaterials Acquisition and Curation Office, Johnson Space Center, NASA, Houston, TX 77058; iDepartment of Civil, Environmental and Mechanical Engineering, University of Trento, I-38123 Trento, Italy; jSaudi Aramco R&D Center, 31311 Dhahran, Saudi Arabia; kSwiss Light Source, Paul Scherrer Institut, 5232 Villigen, Switzerland; lCarl Sagan Center, SETI Institute, Mountain View, CA 94043; and mDepartment of Physics and Astronomy, University of Khartoum, 11111 Khartoum, Sudan Edited by Mark Thiemens, University of California San Diego, La Jolla, CA, and approved August 12, 2020 (received for review October 31, 2019) The origin of diamonds in ureilite meteorites is a timely topic in to various degrees and in these samples the graphite areas, though planetary geology as recent studies have proposed their formation still having external blade-shaped morphologies, are internally at static pressures >20 GPa in a large planetary body, like diamonds polycrystalline (18).