Semi-Annual Technical Report the FLOW of PLASMA in the SOLAR
Total Page:16
File Type:pdf, Size:1020Kb
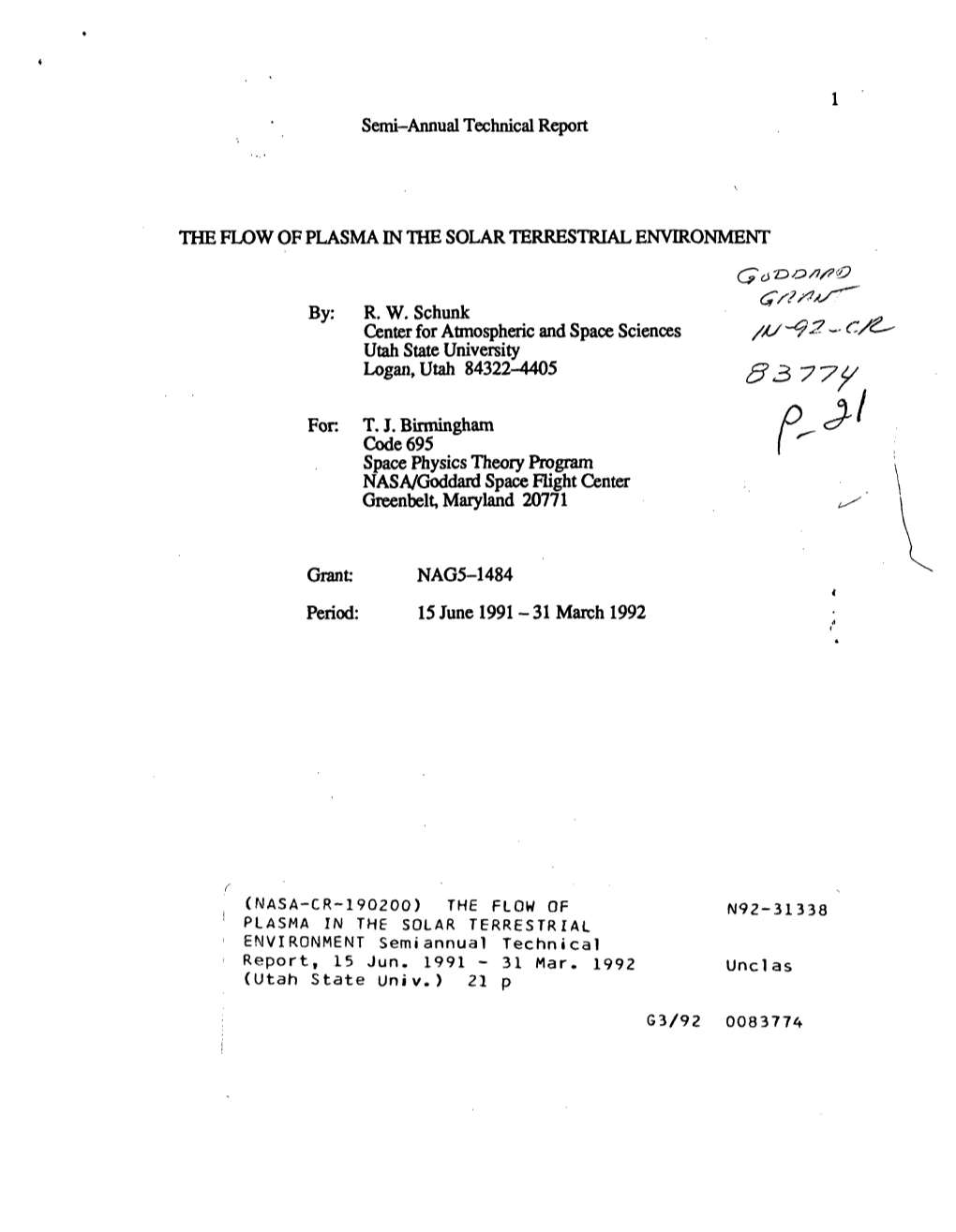
Load more
Recommended publications
-
Planetary Wave Signatures in the Equatorial Atmosphere–Ionosphere System, and Mesosphere- E- and F-Region Coupling
ARTICLE IN PRESS Journal of Atmospheric and Solar-Terrestrial Physics 68 (2006) 509–522 www.elsevier.com/locate/jastp Planetary wave signatures in the equatorial atmosphere–ionosphere system, and mesosphere- E- and F-region coupling M.A. Abdua,Ã, T.K. Ramkumarb, I.S. Batistaa, C.G.M. Bruma, H. Takahashia, B.W. Reinischc, J.H.A. Sobrala aNational Institute for Space Research—INPE, Sao Jose dos Campos, 12245-970 SP, Brazil bNational MST Radar Facility, Gadanki, India cUniversity of Massachusetts, Lowell, USA Available online 8 November 2005 Abstract Upward transport of wave energy and momentum due to gravity, tidal and planetary waves from below and extra- tropics controls the phenomenology of the equatorial atmosphere–ionosphere system. An important aspect of this phenomenology is the development of small- and large-scale structures including thin layers in the mesosphere and E- region, and the formation of wide spectrum plasma structures of the equatorial F-region, widely known as equatorial spread F/plasma bubble irregularities (that are known to have significant impact on space application systems based on trans-ionospheric radio waves propagation). It seems that the effects of tidal and gravity waves at mesospheric and thermospheric heights and their control of ionospheric densities, electric fields and currents are relatively better known than are the effects originating from vertical coupling due to planetary waves. Results from airglow, radar and ionospheric sounding observations demonstrate the existence of significant planetary wave influence on plasma parameters at E- and F- region heights over equatorial latitudes. We present and discuss here some results showing planetary wave oscillations in concurrent mesospheric winds and equatorial electrojet intensity variations in the Indian sector as well as in the mesospheric airglow and F-layer height variation in Brazil. -
Equatorial Ionospheric Response to Different Estimated Disturbed
PUBLICATIONS Journal of Geophysical Research: Space Physics RESEARCH ARTICLE Equatorial Ionospheric Response to Different Estimated 10.1002/2017JA024265 Disturbed Electric Fields as Investigated Using Sheffield Key Points: University Plasmasphere Ionosphere Model at INPE • The equatorial ionosphere response to different disturbed electric fields is M. A. Bravo1,2 , I. S. Batista1 , J. R. Souza1, and A. J. Foppiano3 analyzed using the SUPIM-INPE model • fi We want to nd the best alternative of 1Instituto Nacional de Pesquisas Espaciais, São José dos Campos, São Paulo, Brazil, 2Now at Universidad de Santiago de fi disturbed electric eld, both 3 temporally and spatially, when E × B Chile, Estación Central, Santiago, Chile, Universidad de Concepción, Concepción, Chile measurements are not available • The comparison between simulations and observations will serve to find a Abstract Good ionospheric modeling is important to understand anomalous effects, mainly during hierarchy among the different types of geomagnetic storm events. Ionospheric electric fields, thermospheric winds, and neutral composition are drifts affected at different degrees, depending on the intensity of the magnetic disturbance which, in turns, affects the electron density distribution at all latitudes. The most important disturbed parameter for the equatorial Supporting Information: ionosphere is the electric field, which is responsible for the equatorial ionization anomaly. Here various • Supporting Information S1 electric field measurements and models are analyzed: (1) measured by the Jicamarca incoherent scatter radar Correspondence to: (ISR), (2) from Jicamarca Unattended Long-Term studies of the Ionosphere and Atmosphere (JULIA) radar, 0 M. A. Bravo, (3) deduced from magnetometers, (4) calculated from the time variations of the F layer height (dh F/dt), [email protected] and (5) deduced from interplanetary electric field determinations. -
ESA Space Weather STUDY Alcatel Consortium
ESA Space Weather STUDY Alcatel Consortium SPACE Weather Parameters WP 2100 Version V2.2 1 Aout 2001 C. Lathuillere, J. Lilensten, M. Menvielle With the contributions of T. Amari, A. Aylward, D. Boscher, P. Cargill and S.M. Radicella 1 2 1 INTRODUCTION........................................................................................................................................ 5 2 THE MODELS............................................................................................................................................. 6 2.1 THE SUN 6 2.1.1 Reconstruction and study of the active region static structures 7 2.1.2 Evolution of the magnetic configurations 9 2.2 THE INTERPLANETARY MEDIUM 11 2.3 THE MAGNETOSPHERE 13 2.3.1 Global magnetosphere modelling 14 2.3.2 Specific models 16 2.4 THE IONOSPHERE-THERMOSPHERE SYSTEM 20 2.4.1 Empirical and semi-empirical Models 21 2.4.2 Physics-based models 23 2.4.3 Ionospheric profilers 23 2.4.4 Convection electric field and auroral precipitation models 25 2.4.5 EUV/UV models for aeronomy 26 2.5 METEOROIDS AND SPACE DEBRIS 27 2.5.1 Space debris models 27 2.5.2 Meteoroids models 29 3 THE PARAMETERS ................................................................................................................................ 31 3.1 THE SUN 35 3.2 THE INTERPLANETARY MEDIUM 35 3.3 THE MAGNETOSPHERE 35 3.3.1 The radiation belts 36 3.4 THE IONOSPHERE-THERMOSPHERE SYSTEM 36 4 THE OBSERVATIONS ........................................................................................................................... -
Daytime Dynamo Electrodynamics with Spiral Currents Driven by Strong Winds Revealed by Vapor Trails and Sounding Rocket Probes
UCLA UCLA Previously Published Works Title Daytime Dynamo Electrodynamics With Spiral Currents Driven by Strong Winds Revealed by Vapor Trails and Sounding Rocket Probes. Permalink https://escholarship.org/uc/item/2386m69r Journal Geophysical research letters, 47(15) ISSN 0094-8276 Authors Pfaff, R Larsen, M Abe, T et al. Publication Date 2020-08-03 DOI 10.1029/2020gl088803 Peer reviewed eScholarship.org Powered by the California Digital Library University of California RESEARCH LETTER Daytime Dynamo Electrodynamics With Spiral Currents 10.1029/2020GL088803 Driven by Strong Winds Revealed by Vapor Trails and Key Points: • Comprehensive observations of the Sounding Rocket Probes daytime Sq dynamo electrodynamics R. Pfaff1 , M. Larsen2 , T. Abe3 , H. Habu3, J. Clemmons4 , H. Freudenreich1, D. Rowland1, have been gathered for the first time 5,6 7 8 8 9 • Observed daytime winds in the T. Bullett ,M.‐Y. Yamamoto , S. Watanabe , Y. Kakinami , T. Yokoyama , dynamo region are much larger than J. Mabie5,6 , J. Klenzing1 , R. Bishop10, R. Walterscheid10 , M. Yamamoto9 , expected yet their currents are Y. Yamazaki11 , N. Murphy12, and V. Angelopoulos13 reduced by those of DC electric fields • Winds and currents exhibit an 1NASA Goddard Space Flight Center, Greenbelt, MD, USA, 2Department of Physics and Astronomy, Clemson University, interleaved spiral pattern indicative 3 4 of tidal forcing Clemson, SC, USA, Japan Aerospace Exploration Agency, Tokyo, Japan, Department of Physics and Astronomy, University of New Hampshire, Durham, NH, USA, 5Cooperative -
The Near-Earth Plasma Environment
Space Sci Rev (2012) 168:23–112 DOI 10.1007/s11214-012-9872-6 The Near-Earth Plasma Environment Robert F. Pfaff Jr. Received: 1 March 2012 / Accepted: 3 March 2012 / Published online: 20 June 2012 © US Government 2012 Abstract An overview of the plasma environment near the earth is provided. We describe how the near-earth plasma is formed, including photo-ionization from solar photons and impact ionization at high latitudes from energetic particles. We review the fundamental characteristics of the earth’s plasma environment, with emphasis on the ionosphere and its interactions with the extended neutral atmosphere. Important processes that control iono- spheric physics at low, middle, and high latitudes are discussed. The general dynamics and morphology of the ionized gas at mid- and low-latitudes are described including electro- dynamic contributions from wind-driven dynamos, tides, and planetary-scale waves. The unique properties of the near-earth plasma and its associated currents at high latitudes are shown to depend on precipitating auroral charged particles and strong electric fields which map earthward from the magnetosphere. The upper atmosphere is shown to have profound effects on the transfer of energy and momentum between the high-latitude plasma and the neutral constituents. The article concludes with a discussion of how the near-earth plasma responds to magnetic storms associated with solar disturbances. Keywords Ionosphere · Upper atmosphere · Electric fields 1 Introduction In this article we discuss the near-earth plasma environment, for which the ionosphere is the natural focus. Because this ionized gas co-exists with the neutral atmospheric gas to which it is coupled, the ionized/neutral gas system is more properly considered a partially ionized gas rather than a medium consisting of two distinct, independent fluids. -
The Current State of Ionospheric Wind Dynamo Theory Is Reviewed
J. Geomag. Geoelectr., 31, 287-310, 1979 Ionospheric Wind Dynamo Theory: A Review A. D. RICHMOND SpaceEnvironment Laboratory, National Oceanic and Atmospheric Administration,Boulder, Colorado 80302, U. S. A. (Accepted June 10, 1978) The current state of ionospheric wind dynamo theory is reviewed. Observation- al and theoreticaladvances in recent yearshave permitted more accurate models of the dynamo mechanismto be presentedthan previously,which have lent further credenceto the validity of dynamo theory as the main explanation for quiet-day ionosphericelectric fields and currents at middle and low latitudes. The diurnal component of the wind in the upper E region and lower F region appears to be primarily responsiblefor averagequiet-day currents, although other wind compo- nents give significantcontributions. Observationally,there is a need for better spatial and temporal coverage of wind and electric field data. Theoretically, there is a need for further considerationof the mutual dynamiccoupling among winds, conductivities,electric fields, and electric currents, and for better modeling of nighttimeconditions. 1. Introduction This paper is intended to review the present state of knowledge concerning the theory of the ionospheric wind dynamo. The main emphasis is on current theoreti- cal conceptions, with historical aspects, observational evidence, and treatments of temporal and spatial variability covered more briefly. For further information on dynamo theory and ionospheric currents, previous reviews (K. MAEDA and KATO, 1966; MATSUSHITA, 1967, 1968, 1971a, 1973, 1975, 1977; H. MAEDA, 1968; PRICE, 1969a, b; WAGNER, 1971; AKASOFU and CHAPMAN, 1972; MATSUSHITA and MOZER, 1973; VOLLAND, 1974a; FATKULLIN, 1975; KANE, 1976) will be found useful. 2. Formulation of Dynamo Theory The basic features of ionospheric wind dynamo theory can be formulated as follows. -
Electrodynamics of the Martian Dynamo Region Near Magnetic
GeophysicalResearchLetters RESEARCH LETTER Electrodynamics of the Martian dynamo region 10.1002/2013GL059130 near magnetic cusps and loops Key Points: Jeremy A. Riousset1, Carol S. Paty1, Robert J. Lillis2, Matthew O. Fillingim2, Scott L. England2, • The dynamo current forms in a Paul G. Withers3, and John P.M. Hale1 torus shape due to the E⃗× B⃗ drift of electrons 1School of Earth and Atmospheric Sciences, Georgia Institute of Technology, Atlanta, Georgia, USA, 2Space Sciences • Asymmetries in the electric field Laboratory, University of California, Berkeley, California, USA, 3Astronomy Department, Boston University, Boston, are due to the collision-driven ion dynamics Massachusetts, USA • Magnetized region can alter charge carrier motion from lower to upper atmosphere Abstract Strong and inhomogeneous remanent magnetization on Mars results in a complex pattern of crustal magnetic fields. The geometry and topology of these fields lead to atmospheric electrodynamic Supporting Information: structures that are unique among the bodies of the solar system. In the atmospheric dynamo region • Readme (∼100−250 km altitude), ions depart from the gyropath due to collisions with neutral particles, while • Figure S1 electron motion remains governed by electromagnetic drift. This differential motion of the charge • Figure S2 • Text S1 carriers generates electric currents, which induce a perturbation field. The electromagnetic changes ultimately alter the behavior of the local ionosphere beyond the dynamo region. Here we use multifluid Correspondence to: modeling to investigate the dynamics around an isolated magnetic cusp and around magnetic loops or J. A. Riousset, arcades representative of the magnetic topology near, for example, Terra Sirenum. Our results show [email protected] consistent, circular patterns in the electric current around regions with high local field strength, with possible consequences on atmospheric escape of charged particles. -
Pre-19Th Century... Linking the Aurora with Terrestrial Magnetism... 1200
Historical Milestones in Solar-Terrestrial Physics Pre-19th century... Linking the aurora with terrestrial magnetism... 1200 - compasses already in widespread use 1500s - observations of magnetic declination and dip angles 1600 - William Gilbert, in "de Magnete", postulates that Earth itself is a giant magnet 1610 - Johannes and David Fabricius first make telescopic observations of sunspots 1619 - Galileo Galilei first uses the term "aurora borealis" or "dawn of the north" [1645-1700 - Maunder minimum; anomalously few sunspots or auroras seen] 1716 - Edmond Halley—who had spent years sailing the North and South Atlantic Ocean to map out Earth's magnetic field—points out that the aurora is aligned with the terrestrial magnetic field. (But earlier, in an attempt to explain his magnetic observations, he had speculated that the Earth is composed of several concentric, spherical shells filled with glowing gas, and that auroras are composed of escaping subsurface gas.) 1722 - George Graham is the first to point out continual compass variations 1741 - Olof Hiorter makes systematic observations of the geomagnetic field in fixed locations over time; discovers diurnal variation of field, notes change in Earth's magnetic field as the aurora passes overhead 1770 - aurora australis sighted by Capt. James Cook 1790 - Henry Cavendish estimates auroral height at 52-71 miles by triangulation 19th century... Linking the Sun with auroras and terrestrial magnetism... 1850 - Heinrich Schwabe discovers sunspot cycle 1851 - Edward Sabine connects intensity -
General Disclaimer One Or More of the Following Statements May Affect
https://ntrs.nasa.gov/search.jsp?R=19690023132 2020-03-12T05:03:55+00:00Z General Disclaimer One or more of the Following Statements may affect this Document This document has been reproduced from the best copy furnished by the organizational source. It is being released in the interest of making available as much information as possible. This document may contain data, which exceeds the sheet parameters. It was furnished in this condition by the organizational source and is the best copy available. This document may contain tone-on-tone or color graphs, charts and/or pictures, which have been reproduced in black and white. This document is paginated as submitted by the original source. Portions of this document are not fully legible due to the historical nature of some of the material. However, it is the best reproduction available from the original submission. Produced by the NASA Center for Aerospace Information (CASI) M4C ,,,, 7e, Akoptan, A.V. '' of sutochnot pretsfo t* geomagnitnogo dipolia v sozdanit Sq vartatsii." Akade_ miilar Nauk SSSR. Sibirskoe Ot- delente. Sibirskogo Instituta Zemnogo Magnettzma Ionosferi_i Rasprostranentia Radiovoln.Izvestika No. 1, 84-89 (1966). THE EFFECT OF DAILY (24 HOUR) PRECESSION OF THE GEOMAGNETIC DI- POLE ON THE CREATION OF Sq-VARIATIONS All, by A.V. Akoplan 1. It is a known fact that regular quiet sun-daily variations of the magnetic field of the Earth (Sq) are effected by the sys- tems of electrical currents in the near-Earth space. It is a generally accepted concept that these currents can be found in the thin spherical E-layer of the ionosphere and are created due to the atmospheric dynamo effect. -
2011 Cedar IT Workshop Cover
CEDAR-GEM Joint Workshop Santa Fe, New Mexico June 19 – June 24, 2016 CEDAR IT Poster Session Booklet Tuesday, June 21, 2016 Table of Contents Equatorial Thermosphere or Ionosphere EQIT-01 Kassamba Abdel Aziz Diaby, Estimating equatorial daytime vertical ExB drift velocities from magnetic field variations…………………………………………1 EQIT-02 Jonathon Smith, Global Distribution and Characteristics of Equatorial Plasma Bubbles………………………………………...………………………………....1 EQIT-03 Matthew Young, Parametric Wave Growth in a Hybrid PIC/Fluid Simulation of the Equatorial E Region ………………………………………………………….1 EQIT-04 Tzu-Wei Fang, Equatorial-PRIMO (Problems Related to Ionospheric Models and Observations) …………………………………………………………………….2 EQIT-05 Tzu-Wei Fang, Quantifying the sources of ionospheric Day-to-day variability …2 EQIT-06 Jiahao Zhong, Long-duration depletion in the topside ionospheric total electron content during the recovery phase of the March 2015 strong storm ……………..3 EQIT-07 Qingyu Zhu, Simulations of vertical ion-drag effect on neutral winds and compositions at low and middle latitudes ………………………………………..3 EQIT-08 Arthur D. Richmond, What drives the electrodynamics of the low-latitude evening ionosphere? ……………………………………………………………...4 EQIT-09 Jong-Min Choi, Periodicity in the occurrence of equatorial plasma bubbles ……4 EQIT-10 Fasil Tesema Kebede, Thermospheric wind and temperature measurements using Fabry-Perot Interferometers (FPI) at equatorial region of Ethiopia. …………….4 EQIT-11 Daniel J. Fisher, Stormtime effects in the thermospheric neutral winds and temperatures over Brazil …………………………………………………………5 EQIT-12 Pablo Reyes, Probing the equatorial ionospheric valley region fluctuations with Jicamarca ISR and VIPIR ionosonde …………………………………………….5 EQIT-13 Dustin A. Hickey, Airglow observations of the ionosphere from three imagers in South America …………………………………………………………………...5 EQIT-14 Sovit Khadka, Contribution of Neutral Wind and Electrojet to the Structural Dynamics of Equatorial Ionization Anomaly ……………………………………6 Irregularities of Ionosphere or Atmosphere IRRI-01 Victoriya V. -
Sudden Commencements in the X and Y Fields at Equatorial Stations in The
Earth Planets Space, 59, 397–400, 2007 Sudden commencements in the X and Y fields at equatorial stations in the African sector R. G. Rastogi Physical Research Laboratory, Ahmedabad 380009, India (Received December 26, 2005; Revised June 27, 2006; Accepted July 7, 2006; Online published June 8, 2007) This paper describes the mean direction of the H vector due to Sq (solar quiet) and SC (sudden commencement) at equatorial stations Addis-Ababa (geographical longitude: 39◦E) and at M’Bour (17◦W). The Sq (H) vector aligns fairly well with the direction of the dip declination (D), and the SC (H) vector aligns along the dipole declination () minus the dip declination D. At M’Bour, which is situated in the Atlantic Anomaly region, both the Sq (H) and the SC (H) vectors were along 32◦W of N, while the dip as well as the dipole declinations were 9◦W. Thus, the normal and the disturbance current in the Atlantic region is modulated by some additional meridian currents, resulting in a large increase in the westward Y, the source of which cannot be currently estimated from only one station, M’Bour. This problem requires further investigation. Key words: Equatorial sudden commencements, meridional electrojet current. 1. Introduction aligned with respect to the geographic coordinate system. Solar flares are characterized by a temporary enhance- These are: (1) the northward field, X; (2) the eastward ment of solar UV, X-rays and other types of radiation, all of field, Y; (3) the horizontal field, H, which is equal to (X2 which cause a temporary increase of ionization in the iono- +Y2)1/2. -
The MAGDAS Project the Past and Next 10 Years
1 The MAGDAS project The past and next 10 years A. Yoshikawa and A. Fujimoto, ICSWSE, Kyushu University For Space environment monitoring Magnetically Why global network observations of quiet day geomagnetic field disturbances? ・Visualization of Space weather map from global magnetic field data ×観測点 Start of magnetic storm Magnetic field variations on the ground ・space environment monitoring by using space weather index are generated by reflecting (1 )「solar terrestrial coupling」 electromagnetic disturbances We can investigate across the ・ magnetosphere and ionosphere influences to Earth system caused solar eruptions (2 )「horizontal coupling 」 ・relation between solar activity and electromagnetic energy is climate phenomena and/or climate change distributed from ・global circulation of energy and polar to equatorial ionosphere material of upper atmosphere (3 )「atmospheric vertical coupling」 ・relations between seismic activity propagation of lower atmospheric and solar activity? disturbances to upperこれらを同時に且つ広域に調査できるデータは磁場変動データのみ atmosphere via Super Multipointgravity Geomagnetic wave and field tidal Network waves Observation :important tool for exploring of frontier of solar terrestrial coupling process 4 Recent update of MAGDAS stations (2015-2017) (Enhancement of Equatorial Network : developed 15 new sites) 2015 Tingo Maria@Peru , December (New!) Tarapoto@Peru , December (New!) Colombo@Sri Lanka , February (New!) 2016 Wadena, Canada, September (replace of instrument) Perak@Malaysia , October (New!) Banting@Malaysia , October (New!) 2017