Dissertation RJ Final.Pdf
Total Page:16
File Type:pdf, Size:1020Kb
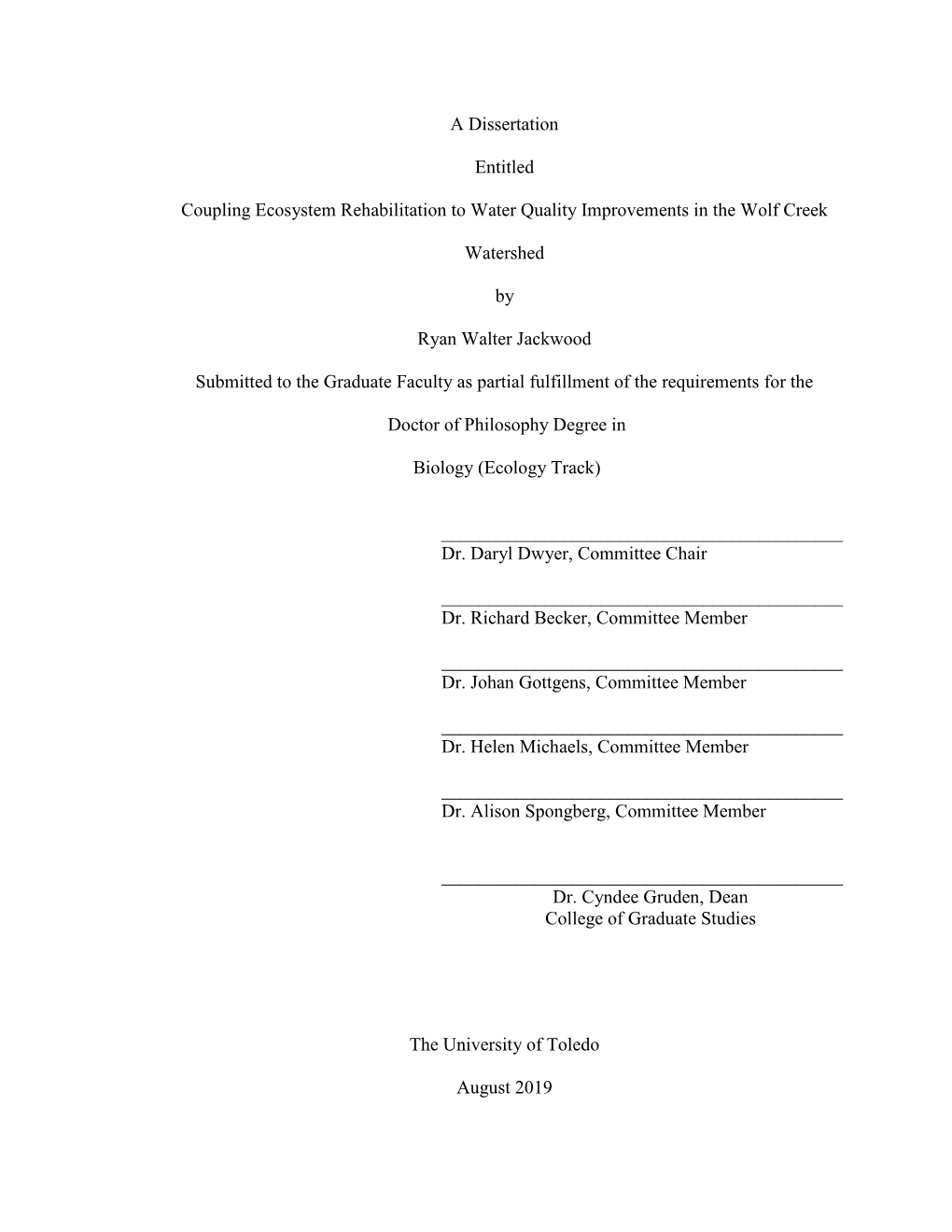
Load more
Recommended publications
-
Evaluating Beneficial Use: Recreation
Ohio 2010 Integrated Report Section F Evaluating Beneficial Use: Recreation F1. Background Prior to the 2002 Integrated Report (IR), the reporting of recreation use impairment in Ohio was sporadic. Section 305(b) reports (1998 and earlier) may have included an indication of the potential for recreation use impairment in various streams, but a cohesive listing was not presented. The 2002 IR employed a uniform methodology to examine readily available data on fecal coliform counts. This approach was based on counting the number of exceedances of the secondary contact recreation use maximum criterion [5000 colony forming units (cfu)/100 ml fecal coliform or 576 cfu/100 ml Escherichia coli (E. coli)]. Any assessment unit with five or more samples over the last five years above these values was listed as having an impaired recreation use. The 2004 IR adopted a more statistically robust methodology for assessing the recreation use attainment of the State’s surface waters linked more directly to the applicable water quality standards. The methodology adopted in 2004 continued to be used through the 2008 IR. The 2008 IR also included a preview of changes anticipated at the time for the 2010 report based on the expectation that the watershed assessment unit (WAU) would change from a larger watershed size (11-digit hydrologic unit) to a smaller watershed size (12-digit hydrologic unit) and on four anticipated revisions to the water quality standards: 1) dropping the fecal coliform criteria; 2) creation of a tiered set of classes of primary contact recreation waters based on recreation use intensity; 3) revision of the geometric mean averaging period; and 4) extension of the recreation season. -
Devoted to the Study and Appreciation of Ohio's Birdlife • Vol. 43, No. 3, Spring 2020
Devoted to the Study and Appreciation of Ohio’s Birdlife • Vol. 43, No. 3, Spring 2020 An exciting find for Gautam Apte, this fledgling Eastern Screech-Owl posed at Shaker Lakes,Cuyahoga , on 08 May. On the cover: The first state record Hooded Oriole was the highlight of spring migration for Bruce Miller, who masterfully photographed it at a private residence in Columbus on 02 April. Vol. 43 No. 3 Devoted to the Study and Appreciation of Ohio’s Birdlife EDITOR OHIO BIRD RECORDS Craig Caldwell COMMITTEE 1270 W. Melrose Dr. Westlake, OH 44145 Jay G. Lehman 440-356-0494 Secretary [email protected] 7064 Shawnee Run Rd. Cincinnati, OH 45243 [email protected] PHOTO EDITOR Jamie Cunningham PAST PUBLISHERS [email protected] John Herman (1978–1980) Edwin C. Pierce (1980–2008) LAYOUT Roger Lau PAST EDITORS [email protected] John Herman (1978–1980) Edwin C. Pierce (1980–1991) Thomas Kemp (1987–1991) CONSULTANTS Robert Harlan (1991–1996) Ron Canterbury Victor W. Fazio III (1996–1997) Tim Colborn Bill Whan (1997–2008) Bob Dudley Andy Jones (2008–2010) Stefan Gleissberg Jill M. Russell (2010–2012) Rob Harlan Andy Jones Kent Miller Brian Wulker And the 27 eBird county reviewers ISSN 1534-1666 The Ohio Cardinal, Spring 2020 COMMENTS ON THE SEASON By Craig Caldwell al, with some places getting as little as 75% of their usual rainfall, most of the southern half got This issue reaches you with the hope that you between 125 and 200% of the norm with Cin- and your loved ones are healthy and that the cinnati’s 15 inches being triple the usual amount. -
A Week Along the Water: a Vision of the Ohio Lake Erie Waterfront in 2050
A week along the water: A vision of the Ohio Lake Erie waterfront in 2050 Written by David Beach, GreenCityBlueLake Institute For the Green Ribbon Coalition August 2016 The following is a transcript of narration from a virtual reality diary posted to the Ohio Fresh Coast neural net on June 15, 2050. Prologue showed the property tax values and job increases that would result if the state purchased lakefront properties. I can’t wait. I have a whole week to bike along the Ohio Instead of a single row of shoreline properties having Lake Erie Greenway. I’m going from Toledo to Cleveland, enhanced value from being on the water, whole with some short side trips along the way. It will be around communities would increase in value because everyone 200 miles. would be able to enjoy the water. The coalition then led a I’m surprised I’ve never done this before. Thousands of campaign to persuade the state to create a bond fund, people do this ride -- more and more every year. They modeled on the popular Clean Ohio Fund, which was come from all over to experience one of the best earmarked for the purchase of lakefront land. Up to $10 greenway trails in the country. Although I’ve been million per year has been available since 2020, and over involved in Ohio coastal issues for a long time and I’ve the years it has allowed the purchase of hundreds of traveled along many parts of the lakefront, I’ve never properties. The amount of public access has nearly biked such a long stretch at doubled to 120 miles, and once. -
Maumee Bay & Northwest Ohio
EExplorexplore MMaumeeaumee BayBay & NNorthwestorthwest OhioOhio Fantastic Fishing....Page 4 Glorious Colors......Page 6 Hit the Links.............Page 9 Family Fun.............Page 12 Fall Issue 2021 www.presspublications.com Volume 33, No. 3 A ffreeree publicationpublication ofof TheThe PressPress NewspapersNewspapers Oregon on the Bay off ers visitors access to the shoreline of Lake Erie, Maumee Bay State Park, Pearson Metropark, shopping, dining and lodging. Oregon is conveniently located as a gateway to and from Lake Erie, the Lake Erie Islands, Cedar Point and all of the attractions along the way. Th e City of Oregon is a great place to visit, but an even better place to live! Sincerely, Mayor Michael Seferian Pearson Metropark Walking, running and biking paths, paddle boats, fi shing, children’s playgrounds, tennis courts, picnic areas, “Window on Wildlife” Exhibit, and Historic Johlin Cabin. Howard Marsh Metropark 6 miles of water trails for canoeing and kayaking and 5 miles of trails for hiking and bicycling. Howard Marsh is a birding hot spot of over 230 species including many rare and unexpected visitors German American Festival, Oak Shade Grove during migration. Maumee Bay State Park Beaches, Boardwalk, picnic areas, bike paths, view of Lake Erie and Toledo Harbor Lighthouse, indoor/outdoor pools and play areas, Nature Center, Storybook Interactive Nature Trail (1/2 mile), lodge, cabins and restaurant. Coontz Recreation Complex Baseball, soft ball, soccer, skate park, basketball, volleyball, pickleball and fi tness courts, (3) playgrounds, children’s water splash pad and bike trails. Storybook Interactive Nature Trail Rollin’ Food and Farm Market 2021 First Wednesday of each month --Sept 1 and Oct 6 Event located at 2973 Dustin Road (between Isaac Streets Drive & Harbor Drive) South Shore Park Boardwalk on Maumee Bay, fi tness trail, picnic area and children’s playground. -
FFY16 Ohio Nonpoint Source Management Program Annual Report
Ohio EPA Program Summary Nonpoint Source Program September 2016 FY2016 Annual Report Alum Creek Lake Photo by Russ Gibson John Kasich, Governor Mary Taylor, Lt. Governor Craig W. Butler, Director Table of Contents Page Introduction ............................................................................................................................................... 4 Grants Management & Administration .................................................................................................... 5 Section 319(h) Grants Management and Administration ........................................................................ 6 - FY12 Section 319(h) Project Site Visits Completed .................................................................................. 8 - FY13 Section 319(h) Project Site Visits Completed .................................................................................. 8 - FY14 Section 319(h) Project Site Visits Completed .................................................................................. 8 - FY15 Section 319(h) Project Site Visits Completed………………………………………………………………………………8 - FY16 Section 319(h) Project Site Visits Completed .................................................................................. 9 - FY12 Section 319 Subgrants Closed ......................................................................................................... 9 - FY13 Section 319 Subgrants Closed ........................................................................................................ -
Scouting in Ohio
Scouting Ohio! Sipp-O Lodge’s Where to Go Camping Guide Written and Published by Sipp-O Lodge #377 Buckeye Council, Inc. B.S.A. 2009 Introduction This book is provided as a reference source. The information herein should not be taken as the Gospel truth. Call ahead and obtain up-to-date information from the place you want to visit. Things change, nothing is guaranteed. All information and prices in this book were current as of the time of publication. If you find anything wrong with this book or want something added, tell us! Sipp-O Lodge Contact Information Mail: Sipp-O Lodge #377 c/o Buckeye Council, Inc. B.S.A. 2301 13th Street, NW Canton, Ohio 44708 Phone: 330.580.4272 800.589.9812 Fax: 330.580.4283 E-Mail: [email protected] [email protected] Homepage: http://www.buckeyecouncil.org/Order%20of%20the%20Arrow.htm Table of Contents Scout Camps Buckeye Council BSA Camps ............................................................ 1 Seven Ranges Scout Reservation ................................................ 1 Camp McKinley .......................................................................... 5 Camp Rodman ........................................................................... 9 Other Councils in Ohio .................................................................... 11 High Adventure Camps .................................................................... 14 Other Area Camps Buckeye .......................................................................................... 15 Pee-Wee ......................................................................................... -
Escherichia Coli and Suspended Sediment in Berger Ditch at Maumee Bay State Park, Oregon, Ohio, 2006
Escherichia coli and Suspended Sediment in Berger Ditch at Maumee Bay State Park, Oregon, Ohio, 2006 Open-File Report 2007–1244 U.S. Department of the Interior U.S. Geological Survey Cover. Clockwise from upper left— A. Staff gage in Berger Ditch at Maumee Bay State Park, Oregon, Ohio. B. U.S. Geological Survey gage house near Berger Ditch. C. Looking downstream at staff gage in Berger Ditch from Cedar Point Road bridge. D. Automatic sampler at gage house at Berger Ditch, Oregon, Ohio (station number 04194085). Escherichia coli and Suspended Sediment in Berger Ditch at Maumee Bay State Park, Oregon, Ohio, 2006 By Amie M.G. Brady Open-File Report 2007–1244 U.S. Department of the Interior U.S. Geological Survey U.S. Department of the Interior DIRK KEMPTHORNE, Secretary U.S. Geological Survey Mark D. Myers, Director U.S. Geological Survey, Reston, Virginia: 2007 For product and ordering information: World Wide Web: http://www.usgs.gov/pubprod Telephone: 1-888-ASK-USGS For more information on the USGS--the Federal source for science about the Earth, its natural and living resources, natural hazards, and the environment: World Wide Web: http://www.usgs.gov Telephone: 1-888-ASK-USGS Any use of trade, product, or firm names is for descriptive purposes only and does not imply endorsement by the U.S. Government. Although this report is in the public domain, permission must be secured from the individual copyright owners to reproduce any copyrighted materials contained within this report. Suggested citation: Brady, A.M.G., 2007, Escherichia coli and suspended sediment in Berger Ditch at Maumee Bay State Park, Oregon, Ohio, 2006: U.S. -
Warbler Capital of the World!
SHORESandISLANDS.com Welcome to the Warbler Capital of the World! Lake Erie Shores & Islands-East Lake Erie Shores & Islands-West Sandusky, Ohio Port Clinton, Ohio 419.625.2984, 800.255.3743 419.734.4386, 800.441.1271 East Shores BERLIN HEIGHTS 1. Edison Woods MetroPark 10186 SR 61 (Ceylon Rd.) 419.625.7783, eriemetroparks.org This 1,300-acre, densely-wooded parkland with 550 acres of wetland habitat, 300 acres of restored native grassland, and ½ mile boardwalk is an excellent place to view the spring neotropical migration. Nesting species include blue-winged and yellow warblers, ovenbirds, scarlet tanagers, and rose-breasted grosbeaks. Seasonal, portable restrooms, seven miles of woodland trails and six miles of meadow pathways for hiking, plus bridle trails (bring your own horse). Year-round, daily 8 am-dusk. 2. Hoffman Forest MetroPark 5313 Huff Rd. 419.625.7783, eriemetroparks.org Home to many species of birds, wildlife, plants, and trees. The grassy trails of this 40-acre park wind through meadow, mature forest, and creek habitats. There is seasonal fishing located in Old Woman Creek with a valid fishing license and permit obtained from Erie MetroParks. Year-round, daily 8 am-dusk. BIRMINGHAM 3. Schoepfle Garden Central Shores 11106 Market St. (off SR 113 E) 440.965.7237, 800.526.7275, metroparks.cc/schoepfle_garden.php CASTALIA A 70-acre arboretum and garden containing 20 acres of topiaries, rose gardens, and 8. Castalia Duck Pond perennial and annual flowers. A 50-acre forest with trails and the Vermilion River SR 269, lakeeriebirding.ohiodnr.gov adjacent to arboretum. Garden paths open Apr to Oct, 8 am-8 pm; Nov to early-Apr, This 12-acre pond is at its best from late-fall to early-spring when waterfowl species head 8 am-4:30 pm. -
Where to Go Camping Guide
The where to go camping guide has been put together by the Order of the Arrow and the Outdoor Program Committee to give a list of places units can go for various activities. It contains a list of Camps, parks, and other facilities available within a reasonable distance. There are roughly 200 locations listed. Our hope is that you will use this guide as a reference as you research and plan your upcoming camping and hiking trips and other activities for your unit. Updated June 2018 Page 1 How to use this guide: The list is alphabetical, and each one contains at least one means of contact info. Below the contact info section is a website link, followed by if it has hiking trails, and last is the list of things the location has to offer. There will usually be two locations listed per page, with the document being 100 pages in length. Contact us: If you have any additions or corrections, please email [email protected] with "Where to Go Camping Guide" in the title. We would like to know if you are using this and we want to continue to add information that is useful to you! How to plan a campout: The Adventure Plan (TAP) is a National resource to help units plan and execute a great camping experience for youth. It includes the following • Ideas for outings / activities • Budgets / financial worksheets • Travel options / reservations & permits • Examples including timetables, duty rosters, and more • Equipment lists • Health and Safety information • List of historic trails And more! It has 52 steps, but don’t let that deter you from using this tool. -
Volume XIII, Issue 6 June, 2015
THE SCOOP is also available online at: June, 2015 www.AARVParks.com Volume XIII, Issue 6 Cathedral Palms, CA Hidden Springs, MS Tomorrow’s Stars, OH 35-901 Cathedral Canyon Drive 16 Clyde Rhodus Road 6716 E. National Road Cathedral City, CA 92234 Tylertown, MS 39667 South Charleston, OH 45368 760-324-8244 601-876-4151 937-324-2267 One would assume that during the May was full of crawfish boils, Last month kept us busy with hot Summer months here, there wagon trains, canoe trips, birthday some last-minute projects, would not be much to do in the celebrations and fun in the sun including the installation of new desert. and pools. doors at the shower house and pool house, adding some fish to Not true! While the pace slows and June is here and so is the Dairy the pond and raising the flags at the traffic all but disappears, Palm Festival. Year after year everybody the entrance to the park. Springs and the surrounding areas has fun the first Saturday in June, have made a concerted effort to On Mother’s Day we had a good entice visits during our ‘slow’ time with a brunch featuring fruit, season. yogurt, muffins, and juice for all of Case in point, Restaurant Week is the perfect time to visit your favorite restaurant or try something new, as Gourmet meals for the frugal! chefs prepare delicious 3 course meals for your dining pleasure, Blaine Morrison with his catch priced at $26 or $38. This year, over even the cows! The festival is free as 100 local restaurants are are all of the activities, making it a New flags at our entrance participating, making the event, real treat for families. -
Public Water System Location Site Date Toxin Result Or Waterbody (Ppb) Inland Lake Grand Lake St
Public Water System Location Site Date Toxin Result or Waterbody (ppb) Inland Lake Grand Lake St. Marys Celina PWS Intake January 12, 2011 Microcystins 0.4 Public Water System Celina, City of Finished Water January 12, 2011 Microcystins <0.15 Inland Lake Grand Lake St. Marys Celina PWS Intake January 19, 2011 Microcystins 0.2 Public Water System Celina, City of Finished Water January 19, 2011 Microcystins <0.15 Inland Lake Grand Lake St. Marys Celina PWS Intake January 26, 2011 Microcystins 0.4 Public Water System Celina, City of Finished Water January 26, 2011 Microcystins <0.15 Inland Lake Grand Lake St. Marys Celina PWS Intake February 3, 2011 Microcystins 0.6 Public Water System Celina, City of Finished Water February 3, 2011 Microcystins <0.15 Inland Lake Grand Lake St. Marys Celina PWS Intake February 9, 2011 Microcystins 0.3 Public Water System Celina, City of Finished Water February 9, 2011 Microcystins <0.15 Inland Lake Grand Lake St. Marys Celina PWS Intake February 16, 2011 Microcystins 0.6 Public Water System Celina, City of Finished Water February 16, 2011 Microcystins <0.15 Inland Lake Grand Lake St. Marys Celina PWS Intake February 23, 2011 Microcystins 0.3 Public Water System Celina, City of Finished Water February 23, 2011 Microcystins <0.15 Inland Lake Grand Lake St. Marys Celina PWS Intake March 2, 2011 Microcystins 0.4 Public Water System Celina, City of Finished Water March 2, 2011 Microcystins <0.15 Inland Lake Grand Lake St. Marys Celina PWS Intake March 9, 2011 Microcystins 0.3 Public Water System Celina, City of Finished Water March 9, 2011 Microcystins <0.15 Inland Lake Grand Lake St. -
Wolf Creek-Frontal Lake Erie HUC-12 (04100010 07 04) Approved: August 21, 2018
Nine-Element Nonpoint Source Implementation Strategic (NPS-IS) Plan Cedar Creek: Wolf Creek-Frontal Lake Erie HUC-12 (04100010 07 04) Approved: August 21, 2018 Created by: The City of Toledo 348 South Erie Street Toledo, OH 43604 In consultation with: Hull & Associates, Inc. 219 South Erie Street Toledo, OH 43604 This page intentionally left blank Wolf Creek-Frontal Lake Erie HUC-12 (04100010 07 04) ii Table of Contents Acknowledgements ....................................................................................................................................... v Chapter 1: Introduction ................................................................................................................................ 1 1.1 Report Background ............................................................................................................................. 1 1.2 Watershed Profile and History ............................................................................................................ 2 1.3 Public Participation and Involvement ................................................................................................. 3 Chapter 2: Wolf Creek-Frontal Lake Erie HUC-12 Watershed Characterization and Assessment Summary 5 2.1 Summary Watershed Characterization for the Wolf Creek-Frontal Lake Erie HUC-12 ...................... 5 2.1.1 Physical and Natural Features ...................................................................................................... 5 2.1.2 Land Use and Protection .............................................................................................................