Stellar Parameters and Metallicities of Stars Hosting Jovian and Neptunian Mass Planets: a Possible Dependence of Planetary Mass on Metallicity∗
Total Page:16
File Type:pdf, Size:1020Kb
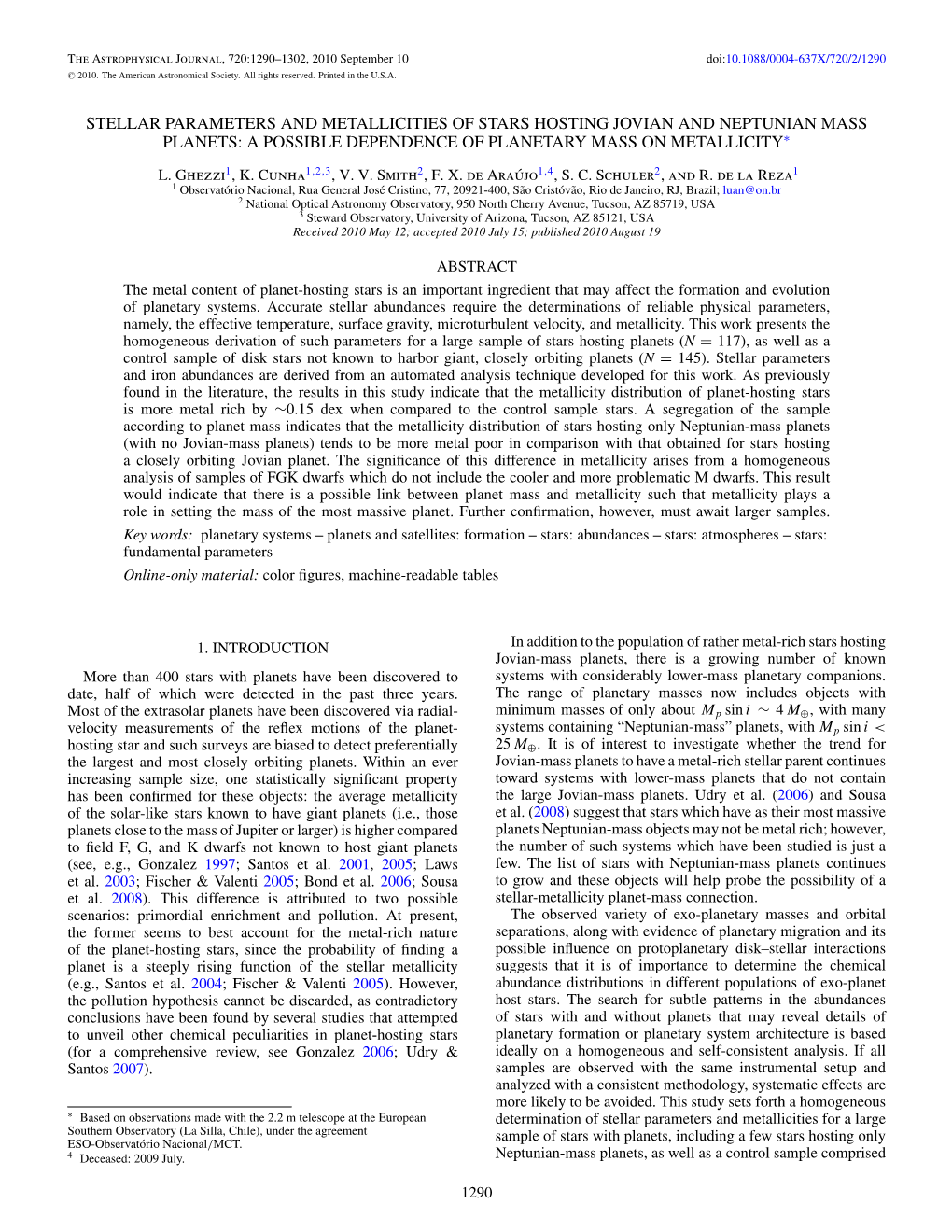
Load more
Recommended publications
-
Lurking in the Shadows: Wide-Separation Gas Giants As Tracers of Planet Formation
Lurking in the Shadows: Wide-Separation Gas Giants as Tracers of Planet Formation Thesis by Marta Levesque Bryan In Partial Fulfillment of the Requirements for the Degree of Doctor of Philosophy CALIFORNIA INSTITUTE OF TECHNOLOGY Pasadena, California 2018 Defended May 1, 2018 ii © 2018 Marta Levesque Bryan ORCID: [0000-0002-6076-5967] All rights reserved iii ACKNOWLEDGEMENTS First and foremost I would like to thank Heather Knutson, who I had the great privilege of working with as my thesis advisor. Her encouragement, guidance, and perspective helped me navigate many a challenging problem, and my conversations with her were a consistent source of positivity and learning throughout my time at Caltech. I leave graduate school a better scientist and person for having her as a role model. Heather fostered a wonderfully positive and supportive environment for her students, giving us the space to explore and grow - I could not have asked for a better advisor or research experience. I would also like to thank Konstantin Batygin for enthusiastic and illuminating discussions that always left me more excited to explore the result at hand. Thank you as well to Dimitri Mawet for providing both expertise and contagious optimism for some of my latest direct imaging endeavors. Thank you to the rest of my thesis committee, namely Geoff Blake, Evan Kirby, and Chuck Steidel for their support, helpful conversations, and insightful questions. I am grateful to have had the opportunity to collaborate with Brendan Bowler. His talk at Caltech my second year of graduate school introduced me to an unexpected population of massive wide-separation planetary-mass companions, and lead to a long-running collaboration from which several of my thesis projects were born. -
An Extreme Planetary System Around HD 219828 One Long-Period Super Jupiter to a Hot-Neptune Host Star?,??
A&A 592, A13 (2016) Astronomy DOI: 10.1051/0004-6361/201628374 & c ESO 2016 Astrophysics An extreme planetary system around HD 219828 One long-period super Jupiter to a hot-Neptune host star?,?? N. C. Santos1; 2, A. Santerne1, J. P. Faria1; 2, J. Rey3, A. C. M. Correia4; 5, J. Laskar5, S. Udry3, V. Adibekyan1, F. Bouchy3; 6, E. Delgado-Mena1, C. Melo7, X. Dumusque3, G. Hébrard8; 9, C. Lovis3, M. Mayor3, M. Montalto1, A. Mortier10, F. Pepe3, P. Figueira1, J. Sahlmann11, D. Ségransan3, and S. G. Sousa1 1 Instituto de Astrofísica e Ciências do Espaço, Universidade do Porto, CAUP, Rua das Estrelas, 4150-762 Porto, Portugal e-mail: [email protected] 2 Departamento de Física e Astronomia, Faculdade de Ciências, Universidade do Porto, Rua do Campo Alegre, 4169-007 Porto, Portugal 3 Observatoire de Genève, Université de Genève, 51 ch. des Maillettes, 1290 Sauverny, Switzerland 4 CIDMA, Departamento de Física, Universidade de Aveiro, Campus de Santiago, 3810-193 Aveiro, Portugal 5 ASD, IMCCE-CNRS UMR 8028, Observatoire de Paris, PSL Research University, 77 Av. Denfert-Rochereau, 75014 Paris, France 6 Aix-Marseille Université, CNRS, Laboratoire d’Astrophysique de Marseille UMR 7326, 13388 Marseille Cedex 13, France 7 European Southern Observatory (ESO), 19001 Casilla, Santiago, Chile 8 Observatoire de Haute-Provence, CNRS/OAMP, 04870 Saint-Michel-l’Observatoire, France 9 Institut d’Astrophysique de Paris, UMR 7095 CNRS, Université Pierre & Marie Curie, 98bis boulevard Arago, 75014 Paris, France 10 SUPA, School of Physics and Astronomy, University of St. Andrews, St. Andrews, KY16 9SS, UK 11 European Space Agency (ESA), Space Telescope Science Institute, 3700 San Martin Drive, Baltimore, MD 21218, USA Received 24 February 2016 / Accepted 10 May 2016 ABSTRACT Context. -