Early Atomic Models – from Mechanical to Quantum (1904-1913)
Total Page:16
File Type:pdf, Size:1020Kb
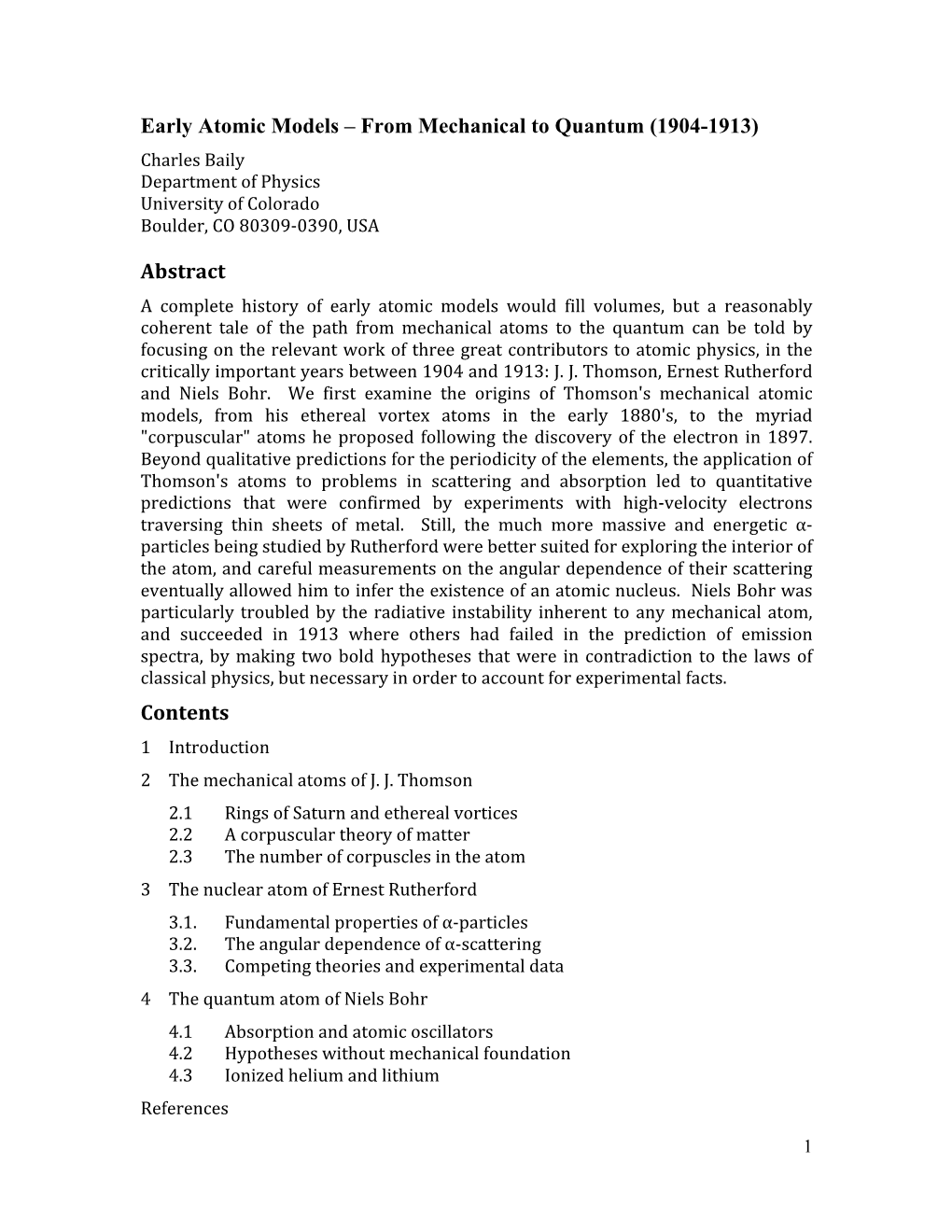
Load more
Recommended publications
-
Atom-Light Interaction and Basic Applications
ICTP-SAIFR school 16.-27. of September 2019 on the Interaction of Light with Cold Atoms Lecture on Atom-Light Interaction and Basic Applications Ph.W. Courteille Universidade de S~aoPaulo Instituto de F´ısicade S~aoCarlos 07/01/2021 2 . 3 . 4 Preface The following notes have been prepared for the ICTP-SAIFR school on 'Interaction of Light with Cold Atoms' held 2019 in S~aoPaulo. They are conceived to support an introductory course on 'Atom-Light Interaction and Basic Applications'. The course is divided into 5 lectures. Cold atomic clouds represent an ideal platform for studies of basic phenomena of light-matter interaction. The invention of powerful cooling and trapping techniques for atoms led to an unprecedented experimental control over all relevant degrees of freedom to a point where the interaction is dominated by weak quantum effects. This course reviews the foundations of this area of physics, emphasizing the role of light forces on the atomic motion. Collective and self-organization phenomena arising from a cooperative reaction of many atoms to incident light will be discussed. The course is meant for graduate students and requires basic knowledge of quan- tum mechanics and electromagnetism at the undergraduate level. The lectures will be complemented by exercises proposed at the end of each lecture. The present notes are mostly extracted from some textbooks (see below) and more in-depth scripts which can be consulted for further reading on the website http://www.ifsc.usp.br/∼strontium/ under the menu item 'Teaching' −! 'Cursos 2019-2' −! 'ICTP-SAIFR pre-doctoral school'. The following literature is recommended for preparation and further reading: Ph.W. -
Taxonomy of Belarusian Educational and Research Portal of Nuclear Knowledge
Taxonomy of Belarusian Educational and Research Portal of Nuclear Knowledge S. Sytova� A. Lobko, S. Charapitsa Research Institute for Nuclear Problems, Belarusian State University Abstract The necessity and ways to create Belarusian educational and research portal of nuclear knowledge are demonstrated. Draft tax onomy of portal is presented. 1 Introduction President Dwight D. Eisenhower in December 1953 presented to the UN initiative "Atoms for Peace" on the peaceful use of nuclear technology. Today, many countries have a strong nuclear program, while other ones are in the process of its creation. Nowadays there are about 440 nuclear power plants operating in 30 countries around the world. Nuclear reac tors are used as propulsion systems for more than 400 ships. About 300 research reactors operate in 50 countries. Such reactors allow production of radioisotopes for medical diagnostics and therapy of cancer, neutron sources for research and training. Approximately 55 nuclear power plants are under construction and 110 ones are planned. Belarus now joins the club of countries that have or are building nu clear power plant. Our country has a large scientific potential in the field of atomic and nuclear physics. Hence it is obvious the necessity of cre ation of portal of nuclear knowledge. The purpose of its creation is the accumulation and development of knowledge in the nuclear field as well as popularization of nuclear knowledge for the general public. *E-mail:[email protected] 212 Wisdom, enlightenment Figure 1: Knowledge management 2 Nuclear knowledge Since beginning of the XXI century the International Atomic Energy Agen cy (IAEA) gives big attention to the nuclear knowledge management (NKM) [1]-[3] . -
Rutherford's Atomic Model
CHAPTER 4 Structure of the Atom 4.1 The Atomic Models of Thomson and Rutherford 4.2 Rutherford Scattering 4.3 The Classic Atomic Model 4.4 The Bohr Model of the Hydrogen Atom 4.5 Successes and Failures of the Bohr Model 4.6 Characteristic X-Ray Spectra and Atomic Number 4.7 Atomic Excitation by Electrons 4.1 The Atomic Models of Thomson and Rutherford Pieces of evidence that scientists had in 1900 to indicate that the atom was not a fundamental unit: 1) There seemed to be too many kinds of atoms, each belonging to a distinct chemical element. 2) Atoms and electromagnetic phenomena were intimately related. 3) The problem of valence (원자가). Certain elements combine with some elements but not with others, a characteristic that hinted at an internal atomic structure. 4) The discoveries of radioactivity, of x rays, and of the electron Thomson’s Atomic Model Thomson’s “plum-pudding” model of the atom had the positive charges spread uniformly throughout a sphere the size of the atom with, the newly discovered “negative” electrons embedded in the uniform background. In Thomson’s view, when the atom was heated, the electrons could vibrate about their equilibrium positions, thus producing electromagnetic radiation. Experiments of Geiger and Marsden Under the supervision of Rutherford, Geiger and Marsden conceived a new technique for investigating the structure of matter by scattering particles (He nuclei, q = +2e) from atoms. Plum-pudding model would predict only small deflections. (Ex. 4-1) Geiger showed that many particles were scattered from thin gold-leaf targets at backward angles greater than 90°. -
Canadian-Built Laser Chills Antimatter to Near Absolute Zero for First Time Researchers Achieve World’S First Manipulation of Antimatter by Laser
UNDER EMBARGO UNTIL 8:00 AM PT ON WED MARCH 31, 2021 DO NOT DISTRIBUTE BEFORE EMBARGO LIFT Canadian-built laser chills antimatter to near absolute zero for first time Researchers achieve world’s first manipulation of antimatter by laser Vancouver, BC – Researchers with the CERN-based ALPHA collaboration have announced the world’s first laser-based manipulation of antimatter, leveraging a made-in-Canada laser system to cool a sample of antimatter down to near absolute zero. The achievement, detailed in an article published today and featured on the cover of the journal Nature, will significantly alter the landscape of antimatter research and advance the next generation of experiments. Antimatter is the otherworldly counterpart to matter; it exhibits near-identical characteristics and behaviours but has opposite charge. Because they annihilate upon contact with matter, antimatter atoms are exceptionally difficult to create and control in our world and had never before been manipulated with a laser. “Today’s results are the culmination of a years-long program of research and engineering, conducted at UBC but supported by partners from across the country,” said Takamasa Momose, the University of British Columbia (UBC) researcher with ALPHA’s Canadian team (ALPHA-Canada) who led the development of the laser. “With this technique, we can address long-standing mysteries like: ‘How does antimatter respond to gravity? Can antimatter help us understand symmetries in physics?’. These answers may fundamentally alter our understanding of our Universe.” Since its introduction 40 years ago, laser manipulation and cooling of ordinary atoms have revolutionized modern atomic physics and enabled several Nobel-winning experiments. -
Research Group of the Committee for the Publication of Hantaro Nagaoka's Biography
Research Group of the Committee for the Publication of Hantaro Nagaoka's Biography Eri Yagi* The Research Group, whose members are Dr. Kiyonobu Itakura of the Na tional Institute for Education Research, Mr. Tosaku Kimura of the National Science Museum, and myself, has completed a biography of Hantaro Nagaoka, which will be published soon (in Japanese) by the Asahi Newspaper Publisher in Tokyo. The Research Group was organized by the Committee in 1963. It was just after the special exhibition of Nagaoka's science activities, held at the National Science Museum in Tokyo by the support of the History of Science Society of Japan. The Committee has been directed by Professor Yoshio Fujioka, who had learned physics under Nagaoka. Unpublished materials, e.g., Nagaoka's notebooks, diaries, corespondences, photos were generosly donated to the National Science Museum by the family of Nagaoka. In addition, those who had been in contact with Nagaoka kindly con tributed informations to the Research Group. The above materials and informations have been arranged, cataloged, and examined by the Research Group. Hantaro Nagaoka was bom at Nagasaki prefecture in the southern part of Japan in 1865 and died in Tokyo in 1950. He was primarily responsible for pro moting the advancement of physics in Japan, between 1900 and 1925, as a professor at the Department of Physics, the University of Tokyo. In the earlier period before Nagaoka started his researches, such local studies as the properties of Japanese magic mirrors, earthquakes, and geomagnetism had dominated by the influence of foreign teachers in Japan. In addition to the study of atomic structure, Nagaoka covered varied fields in physics as magnetostriction, geophysics, mathematical physics, spectroscopy, and radio waves. -
Atomic Physicsphysics AAA Powerpointpowerpointpowerpoint Presentationpresentationpresentation Bybyby Paulpaulpaul E.E.E
ChapterChapter 38C38C -- AtomicAtomic PhysicsPhysics AAA PowerPointPowerPointPowerPoint PresentationPresentationPresentation bybyby PaulPaulPaul E.E.E. Tippens,Tippens,Tippens, ProfessorProfessorProfessor ofofof PhysicsPhysicsPhysics SouthernSouthernSouthern PolytechnicPolytechnicPolytechnic StateStateState UniversityUniversityUniversity © 2007 Objectives:Objectives: AfterAfter completingcompleting thisthis module,module, youyou shouldshould bebe ableable to:to: •• DiscussDiscuss thethe earlyearly modelsmodels ofof thethe atomatom leadingleading toto thethe BohrBohr theorytheory ofof thethe atom.atom. •• DemonstrateDemonstrate youryour understandingunderstanding ofof emissionemission andand absorptionabsorption spectraspectra andand predictpredict thethe wavelengthswavelengths oror frequenciesfrequencies ofof thethe BalmerBalmer,, LymanLyman,, andand PashenPashen spectralspectral series.series. •• CalculateCalculate thethe energyenergy emittedemitted oror absorbedabsorbed byby thethe hydrogenhydrogen atomatom whenwhen thethe electronelectron movesmoves toto aa higherhigher oror lowerlower energyenergy level.level. PropertiesProperties ofof AtomsAtoms ••• AtomsAtomsAtoms areareare stablestablestable andandand electricallyelectricallyelectrically neutral.neutral.neutral. ••• AtomsAtomsAtoms havehavehave chemicalchemicalchemical propertiespropertiesproperties whichwhichwhich allowallowallow themthemthem tototo combinecombinecombine withwithwith otherotherother atoms.atoms.atoms. ••• AtomsAtomsAtoms emitemitemit andandand absorbabsorbabsorb -
1 CHEM 1411 Chapter 5 Homework Answers 1. Which Statement Regarding the Gold Foil Experiment Is False?
1 CHEM 1411 Chapter 5 Homework Answers 1. Which statement regarding the gold foil experiment is false? (a) It was performed by Rutherford and his research group early in the 20th century. (b) Most of the alpha particles passed through the foil undeflected. (c) The alpha particles were repelled by electrons (d) It suggested the nuclear model of the atom. (e) It suggested that atoms are mostly empty space. 2. Ernest Rutherford's model of the atom did not specifically include the ___. (a) neutron (b) nucleus (c) proton (d) electron (e) electron or the proton 3. The gold foil experiment suggested ___. (a) that electrons have negative charges (b) that protons have charges equal in magnitude but opposite in sign to those of electrons (c) that atoms have a tiny, positively charged, massive center (d) the ratio of the mass of an electron to the charge of an electron (e) the existence of canal rays 4. Which statement is false? (a) Ordinary chemical reactions do not involve changes in nuclei. (b) Atomic nuclei are very dense. (c) Nuclei are positively charged. (d) Electrons contribute only a little to the mass of an atom (e) The nucleus occupies nearly all the volume of an atom. 2 5. In interpreting the results of his "oil drop" experiment in 1909, ___ was able to determine ___. (a) Robert Millikan; the charge on a proton (b) James Chadwick; that neutrons are also present in the nucleus (c) James Chadwick; that the masses of protons and electrons are nearly identical (d) Robert Millikan; the charge on an electron (e) Ernest Rutherford; the extremely dense nature of the nuclei of atoms 6. -
Manipulating Atoms with Photons
Manipulating Atoms with Photons Claude Cohen-Tannoudji and Jean Dalibard, Laboratoire Kastler Brossel¤ and Coll`ege de France, 24 rue Lhomond, 75005 Paris, France ¤Unit¶e de Recherche de l'Ecole normale sup¶erieure et de l'Universit¶e Pierre et Marie Curie, associ¶ee au CNRS. 1 Contents 1 Introduction 4 2 Manipulation of the internal state of an atom 5 2.1 Angular momentum of atoms and photons. 5 Polarization selection rules. 6 2.2 Optical pumping . 6 Magnetic resonance imaging with optical pumping. 7 2.3 Light broadening and light shifts . 8 3 Electromagnetic forces and trapping 9 3.1 Trapping of charged particles . 10 The Paul trap. 10 The Penning trap. 10 Applications. 11 3.2 Magnetic dipole force . 11 Magnetic trapping of neutral atoms. 12 3.3 Electric dipole force . 12 Permanent dipole moment: molecules. 12 Induced dipole moment: atoms. 13 Resonant dipole force. 14 Dipole traps for neutral atoms. 15 Optical lattices. 15 Atom mirrors. 15 3.4 The radiation pressure force . 16 Recoil of an atom emitting or absorbing a photon. 16 The radiation pressure in a resonant light wave. 17 Stopping an atomic beam. 17 The magneto-optical trap. 18 4 Cooling of atoms 19 4.1 Doppler cooling . 19 Limit of Doppler cooling. 20 4.2 Sisyphus cooling . 20 Limits of Sisyphus cooling. 22 4.3 Sub-recoil cooling . 22 Subrecoil cooling of free particles. 23 Sideband cooling of trapped ions. 23 Velocity scales for laser cooling. 25 2 5 Applications of ultra-cold atoms 26 5.1 Atom clocks . 26 5.2 Atom optics and interferometry . -
Models of the Atom
David Sang Models of the atom Today we are very familiar with the picture of the atom as a particle with a tiny nucleus at its centre and a cloud of electrons orbiting around the nucleus. But where did this model come from? What were scientists trying to explain using their models of the atom? To find out, we have to go back to the early years of the twentieth century. A model of the atom. But does the nucleus glow like this? No. And are electrons blue? No. Discovering radioactivity and the electron By the 1890s, most physicists were convinced that matter was made up of atoms. The idea of vast In this photo, an electron beam is bent along a circular path by a magnetic field. numbers of tiny, moving particles could explain The beam is produced at the bottom in an ‘electron gun’ and follows a clockwise many things, including the behaviour of gases and path inside the evacuated tube. A small amount of gas has been left in the tube; the differences between the chemical elements. this glows to show up the path of the beam. Then, in 1896, Henri Becquerel discovered radioactivity. He was investigating uranium salts, The importance of these two discoveries was that many of which glow in the dark. To his surprise, he they suggested that atoms were not indestructible. Key words Atoms are tiny but they are made of still smaller found that all the uranium-containing substances model that he tested produced invisible radiation that particles. could blacken photographic paper. -
Culturally Inherited Cognitive Activity: Implications for the Rhetoric of Science
University of Windsor Scholarship at UWindsor OSSA Conference Archive OSSA 4 May 17th, 9:00 AM - May 19th, 5:00 PM Culturally Inherited Cognitive Activity: Implications for the Rhetoric of Science Joseph Little Follow this and additional works at: https://scholar.uwindsor.ca/ossaarchive Part of the Philosophy Commons Little, Joseph, "Culturally Inherited Cognitive Activity: Implications for the Rhetoric of Science" (2001). OSSA Conference Archive. 75. https://scholar.uwindsor.ca/ossaarchive/OSSA4/papersandcommentaries/75 This Paper is brought to you for free and open access by the Conferences and Conference Proceedings at Scholarship at UWindsor. It has been accepted for inclusion in OSSA Conference Archive by an authorized conference organizer of Scholarship at UWindsor. For more information, please contact [email protected]. Title: Culturally Inherited Cognitive Activity: Implications for the Rhetoric of Science1 Author: Joseph Little Response to this paper by: Mark Weinstein © 2001 Joseph Little Introduction Few constructs rest more securely upon the foundation of rhetorical theory than the syllogism. Introduced by Aristotle in the fourth century BC, the syllogism provided Athenian orators with structural guidance for constructing valid, deductive arguments in the polis (Aristotle, 1991, 40; Aristotle, 1984a; Aristotle, 1984b). Yet this guidance is not without qualification: Often neglected in modern times is the fact that valid conclusions, for Aristotle, were only expected to follow necessarily from the premises when the audience was comprised of men acting in accordance with orthos logos, or "right reason" (1984c, 1935-36; 1984d, 1766, 1797, 1798, 1808, 1812, 1819). Also neglected in modern times is the fact that Aristotle thought of "right reason" as a developed ability that "comes to us if growth is allowed to proceed regularly" rather than as an innate aspect of human cognition (1984c, 1939-40). -
22. Atomic Physics and Quantum Mechanics
22. Atomic Physics and Quantum Mechanics S. Teitel April 14, 2009 Most of what you have been learning this semester and last has been 19th century physics. Indeed, Maxwell's theory of electromagnetism, combined with Newton's laws of mechanics, seemed at that time to express virtually a complete \solution" to all known physical problems. However, already by the 1890's physicists were grappling with certain experimental observations that did not seem to fit these existing theories. To explain these puzzling phenomena, the early part of the 20th century witnessed revolutionary new ideas that changed forever our notions about the physical nature of matter and the theories needed to explain how matter behaves. In this lecture we hope to give the briefest introduction to some of these ideas. 1 Photons You have already heard in lecture 20 about the photoelectric effect, in which light shining on the surface of a metal results in the emission of electrons from that surface. To explain the observed energies of these emitted elec- trons, Einstein proposed in 1905 that the light (which Maxwell beautifully explained as an electromagnetic wave) should be viewed as made up of dis- crete particles. These particles were called photons. The energy of a photon corresponding to a light wave of frequency f is given by, E = hf ; where h = 6:626×10−34 J · s is a new fundamental constant of nature known as Planck's constant. The total energy of a light wave, consisting of some particular number n of photons, should therefore be quantized in integer multiples of the energy of the individual photon, Etotal = nhf. -
A Computational Study of Ruthenium Metal Vinylidene Complexes: Novel Mechanisms and Catalysis
A Computational Study of Ruthenium Metal Vinylidene Complexes: Novel Mechanisms and Catalysis David G. Johnson PhD University of York, Department of Chemistry September 2013 Scientist: How much time do we have professor? Professor Frink: Well according to my calculations, the robots won't go berserk for at least 24 hours. (The robots go berserk.) Professor Frink: Oh, I forgot to er, carry the one. - The Simpsons: Itchy and Scratchy land Abstract A theoretical investigation into several reactions is reported, centred around the chemistry, formation, and reactivity of ruthenium vinylidene complexes. The first reaction discussed involves the formation of a vinylidene ligand through non-innocent ligand-mediated alkyne- vinylidene tautomerization (via the LAPS mechanism), where the coordinated acetate group acts as a proton shuttle allowing rapid formation of vinylidene under mild conditions. The reaction of hydroxy-vinylidene complexes is also studied, where formation of a carbonyl complex and free ethene was shown to involve nucleophilic attack of the vinylidene Cα by an acetate ligand, which then fragments to form the coordinated carbonyl ligand. Several mechanisms are compared for this reaction, such as transesterification, and through allenylidene and cationic intermediates. The CO-LAPS mechanism is also examined, where differing reactivity is observed with the LAPS mechanism upon coordination of a carbonyl ligand to the metal centre. The system is investigated in terms of not only the differing outcomes to the LAPS-type mechanism, but also with respect to observed experimental Markovnikov and anti-Markovnikov selectivity, showing a good agreement with experiment. Finally pyridine-alkenylation to form 2-styrylpyridine through a half-sandwich ruthenium complex is also investigated.