Sulfur and Oxygen Species from a Pele-Type Eruption
Total Page:16
File Type:pdf, Size:1020Kb
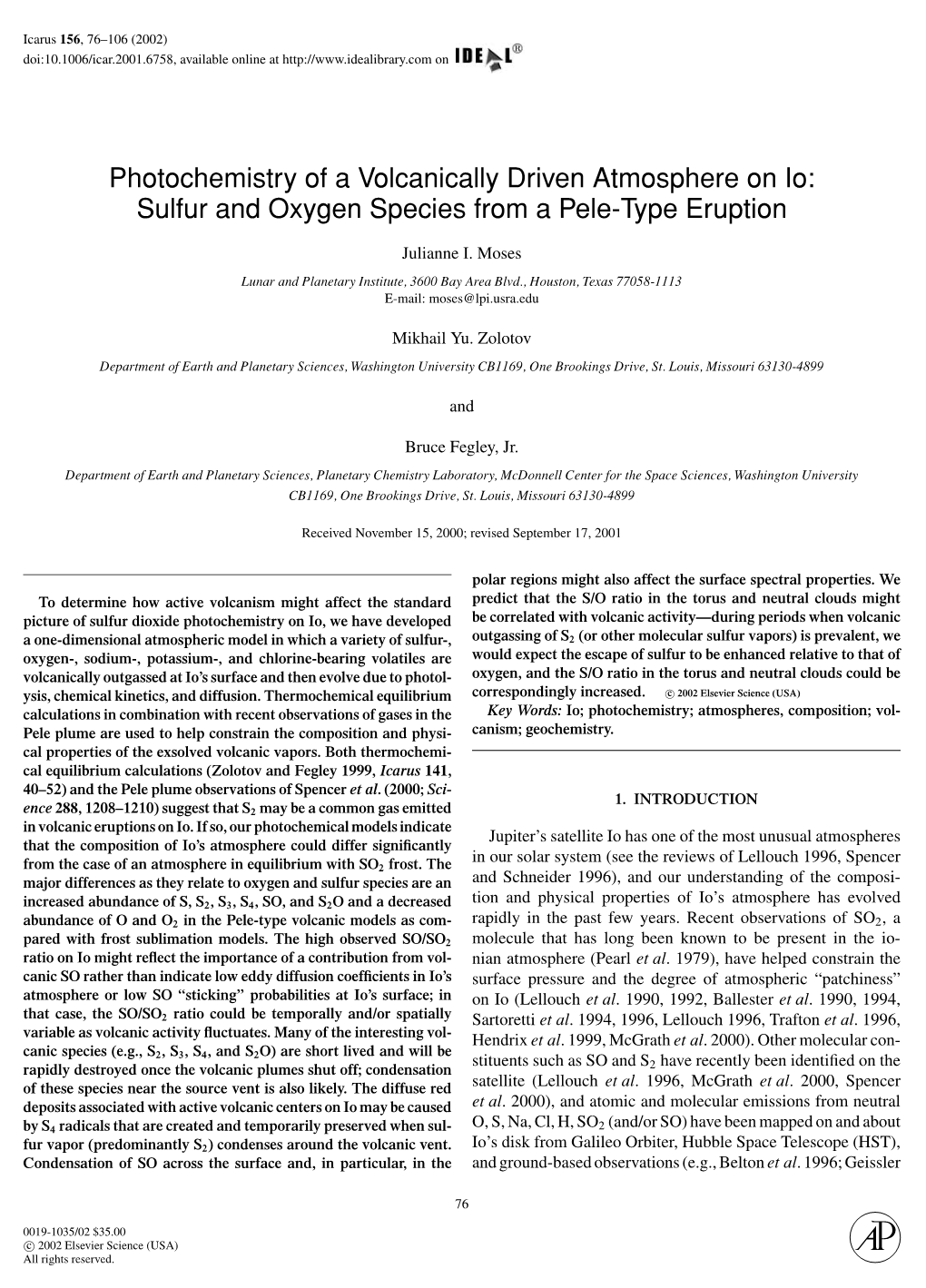
Load more
Recommended publications
-
Appendix 1: Io's Hot Spots Rosaly M
Appendix 1: Io's hot spots Rosaly M. C. Lopes,Jani Radebaugh,Melissa Meiner,Jason Perry,and Franck Marchis Detections of plumes and hot spots by Galileo, Voyager, HST, and ground-based observations. Notes and sources . (N) NICMOS hot spots detected by Goguen etal . (1998). (D) Hot spots detected by C. Dumas etal . in 1997 and/or 1998 (pers. commun.). Keck are hot spots detected by de Pater etal . (2004) and Marchis etal . (2001) from the Keck telescope using Adaptive Optics. (V, G, C) indicate Voyager, Galileo,orCassini detection. Other ground-based hot spots detected by Spencer etal . (1997a). Galileo PPR detections from Spencer etal . (2000) and Rathbun etal . (2004). Galileo SSIdetections of hot spots, plumes, and surface changes from McEwen etal . (1998, 2000), Geissler etal . (1999, 2004), Kezthelyi etal. (2001), and Turtle etal . (2004). Galileo NIMS detections prior to orbit C30 from Lopes-Gautier etal . (1997, 1999, 2000), Lopes etal . (2001, 2004), and Williams etal . (2004). Locations of surface features are approximate center of caldera or feature. References de Pater, I., F. Marchis, B. A. Macintosh, H. G. Rose, D. Le Mignant, J. R. Graham, and A. G. Davies. 2004. Keck AO observations of Io in and out of eclipse. Icarus, 169, 250±263. 308 Appendix 1: Io's hot spots Goguen, J., A. Lubenow, and A. Storrs. 1998. HST NICMOS images of Io in Jupiter's shadow. Bull. Am. Astron. Assoc., 30, 1120. Geissler, P. E., A. S. McEwen, L. Keszthelyi, R. Lopes-Gautier, J. Granahan, and D. P. Simonelli. 1999. Global color variations on Io. Icarus, 140(2), 265±281. -
Atmospheric Escape and the Evolution of Close-In Exoplanets
Atmospheric Escape and the Evolution of Close-in Exoplanets James E. Owen Astrophysics Group, Imperial College London, Blackett Laboratory, Prince Consort Road, London SW7 2AZ, UK; email: [email protected] Xxxx. Xxx. Xxx. Xxx. YYYY. AA:1{26 Keywords https://doi.org/10.1146/((please add atmospheric evolution, exoplanets, exoplanet composition article doi)) Copyright c YYYY by Annual Reviews. Abstract All rights reserved Exoplanets with substantial Hydrogen/Helium atmospheres have been discovered in abundance, many residing extremely close to their par- ent stars. The extreme irradiation levels these atmospheres experience causes them to undergo hydrodynamic atmospheric escape. Ongoing atmospheric escape has been observed to be occurring in a few nearby exoplanet systems through transit spectroscopy both for hot Jupiters and lower-mass super-Earths/mini-Neptunes. Detailed hydrodynamic calculations that incorporate radiative transfer and ionization chemistry are now common in one-dimensional models, and multi-dimensional calculations that incorporate magnetic-fields and interactions with the arXiv:1807.07609v3 [astro-ph.EP] 6 Jun 2019 interstellar environment are cutting edge. However, there remains very limited comparison between simulations and observations. While hot Jupiters experience atmospheric escape, the mass-loss rates are not high enough to affect their evolution. However, for lower mass planets at- mospheric escape drives and controls their evolution, sculpting the ex- oplanet population we observe today. 1 Contents 1. -
The Loss of Nitrogen-Rich Atmospheres from Earth-Like Exoplanets Within M-Star Habitable Zones
**FULL TITLE** ASP Conference Series, Vol. **VOLUME**, **YEAR OF PUBLICATION** **NAMES OF EDITORS** The loss of nitrogen-rich atmospheres from Earth-like exoplanets within M-star habitable zones H. Lammer Space Research Institute, Austrian Academy of Sciences, Schmiedlstr. 6, A-8042 Graz, Austria H. I. M. Lichtenegger Space Research Institute, Austrian Academy of Sciences, Schmiedlstr. 6, A-8042 Graz, Austria M. L. Khodachenko Space Research Institute, Austrian Academy of Sciences, Schmiedlstr. 6, A-8042 Graz, Austria Yu. N. Kulikov Polar Geophysical Institute (PGI), Russian Academy of Sciences, Khalturina Str. 15, Murmansk, 183010, Russian Federation J.-M. Grie¼meier ASTRON, Dwingeloo, The Netherlands Abstract. After the ¯rst discovery of massive Earth-like exoplanets around M-type dwarf stars, the search for exoplanets which resemble more an Earth analogue continues. The discoveries of super-Earth planets pose questions on habitability and the possible origin of life on such planets. Future exoplanet space projects designed to characterize the atmospheres of terrestrial exoplanets will also search for atmospheric species which are considered as bio-markers (e.g. O3,H2O, CH4, etc.). By using the Earth with its atmosphere as a proxy and in agreement with the classical habitable zone concept, one should expect that Earth-like exoplanets suitable for life as we know it should have a nitrogen atmo- sphere and a very low CO2 content. Whether a water bearing terrestrial planet within its habitable zone can evolve into a habitable world similar than the Earth, depends on the capability of its water-inventory and atmosphere to sur- vive the period of high radiation of the young and/or active host star. -
The Origin of Titan's Atmosphere: Some Recent Advances
The origin of Titan’s atmosphere: some recent advances By Tobias Owen1 & H. B. Niemann2 1University of Hawaii, Institute for Astronomy, 2680 Woodlawn Drive, Honolulu, HI 96822, USA 2Laboratory for Atmospheres, Goddard Space Fight Center, Greenbelt, MD 20771, USA It is possible to make a consistent story for the origin of Titan’s atmosphere starting with the birth of Titan in the Saturn subnebula. If we use comet nuclei as a model, Titan’s nitrogen and methane could easily have been delivered by the ice that makes up ∼50% of its mass. If Titan’s atmospheric hydrogen is derived from that ice, it is possible that Titan and comet nuclei are in fact made of the same protosolar ice. The noble gas abundances are consistent with relative abundances found in the atmospheres of Mars and Earth, the sun, and the meteorites. Keywords: Origin, atmosphere, composition, noble gases, deuterium 1. Introduction In this note, we will assume that Titan originated in Saturn’s subnebula as a result of the accretion of icy planetesimals: particles and larger lumps made of ice and rock. Alibert & Mousis (2007) reached this same point of view using an evolutionary, turbulent model of Saturn’s subnebula. They found that planetesimals made in the solar nebula according to their model led to a huge overabundance of CO on Titan. We obviously have no direct measurements of the composition of these planetes- imals. We can use comets as a guide, always remembering that comets formed in the solar nebula where conditions must have been different from those in Saturn’s subnebula, e.g., much colder. -
5 Escape of Atmospheres to Space
5 Escape of Atmospheres to Space So far, our discussion of atmospheric evolution has circumstances: Jeans’ escape, where individual mol- concentrated on atmosphere and climate fundamentals. ecules evaporate into a collisionless exosphere, and Climate constrains possible life and, as we will see later hydrodynamic escape, which is a bulk outflow with a in this book, the way that climate is thought to have velocity driven by atmospheric heating that induces an evolved can explain many environmental differences upward pressure gradient force (e.g., Johnson et al., between Earth, Venus, and Mars. Climate is closely tied 2013d; Walker, 1982). (ii) Suprathermal (or nonthermal) to the composition of a planet’s atmosphere, which deter- escape is where individual atoms or molecules are mines the greenhouse effect. Consequently, to understand boosted to escape velocity because of chemical reactions how climate has changed over time, we must consider or ionic interactions. Finally, (iii) impact erosion is where how atmospheric composition has evolved. In turn, we atmospheric gases are expelled en masse as a result of must examine how atmospheric gases can be lost. large body impacts, such as the cumulative effect of Gases are lost at an atmosphere’s upper and lower asteroids hits. Of these three types, nonthermal escape is boundaries: the planet’s surface and interplanetary space. generally slow because if it were fast the molecules would In this chapter, we consider the latter. Studies of the Solar collide and the escape would be in the thermal category. System have shown that some bodies are vulnerable to Theory suggests that the two mechanisms that can most atmospheric escape (Hunten, 1990). -
Atmospheric Escape from Hot Jupiters
A&A 418, L1–L4 (2004) Astronomy DOI: 10.1051/0004-6361:20040106 & c ESO 2004 Astrophysics Atmospheric escape from hot Jupiters A. Lecavelier des Etangs1, A. Vidal-Madjar1, J. C. McConnell2,andG.H´ebrard1 1 Institut d’Astrophysique de Paris, CNRS, 98bis boulevard Arago, 75014 Paris, France 2 Department of Earth and Atmospheric Science, York University, North York, Ontario, Canada Letter to the Editor Received 10 November 2003 / Accepted 4 March 2004 Abstract. The extra-solar planet HD 209458b has been found to have an extended atmosphere of escaping atomic hydrogen (Vidal-Madjar et al. 2003), suggesting that “hot Jupiters” closer to their parent stars could evaporate. Here we estimate the atmospheric escape (so called evaporation rate) from hot Jupiters and their corresponding life time against evaporation. The calculated evaporation rate of HD 209458b is in excellent agreement with the H Lyman-α observations. We find that the tidal forces and high temperatures in the upper atmosphere must be taken into account to obtain reliable estimate of the atmospheric escape. Because of the tidal forces, we show that there is a new escape mechanism at intermediate temperatures at which the exobase reaches the Roche lobe. From an energy balance, we can estimate plausible values for the planetary exospheric temperatures, and thus obtain typical life times of planets as a function of their mass and orbital distance. Key words. star: individual: HD 209458 – stars: planetary systems 1. Introduction both planets (Chamberlain & Hunten 1987). Although the ob- served high temperatures in the giant planets are not yet ex- Among the more than one hundred extra-solar planets known, plained, extreme and far ultraviolet fluxes, Solar wind and per- over 15% are closer than 0.1 AU from the central star. -
Space Physics at Venus: Exploring Atmospheric Dynamics, Escape, and Evolution
Space Physics at Venus: Exploring atmospheric dynamics, escape, and evolution Team lead and point of Contact: Glyn Collinson (NASA Goddard Space Flight Center / Catholic University of America) Telephone: +1-301-286-2511 Email: [email protected] Co-Authors: Steve Boucher (UMich), Shannon Curry (UC Berkeley), Gina DiBraccio (NASA GSFC), Chuanfei Dong (Princeton University), Chris Fowler (UC Berkeley), Yoshifumi Futaana (IRF, Kiruna), Steve Ledvina (UC Berkeley), Janet Luhmann (UC Berkeley), Robin Ramstad (LASP), Joe O'Rourke (Arizona State), Chris Russell (UCLA), Moa Persson (IRF, Kiruna), Michael Way (Goddard Institute for Space Studies), Shaosui Xu (UC Berkeley) Co-signers with their respective institutions: Kevin Baines (JPL), Amanda Brecht (NASA Ames), Jared Espley (NASA GSFC), Matthew Fillingim (UC Berkeley), Candace Grey (New Mexico State), Kerrin Hensley (Boston University), Robert Herrick (University of Alaska Fairbanks), Noam Izenberg (APL), Emilie Royer (Planetary Science Institute), Constantine Tsang (SWRI), Zach Williams Abstract: Our understanding of ancient Venus and its evolution to the present day could be substantially advanced through future Space Physics investigations from orbit. We outline three high-priority strawman investigations, each possible for relative thrift with existing (or near-future) technology. 1.) To understand the physical processes that facilitate Venusian atmospheric escape to space, so that we may extrapolate backwards through time; 2.) To explore ancient Venus through the measurement of the escape rates of key species such as Deuterium, Noble elements, and Nitrogen; 3.) To understand and quantify how energy and momentum is transferred from the solar wind, through the ionosphere, and into the atmosphere, so that we may reveal its impact to the dynamics of the atmosphere. -
Atmospheric Escape A.J.Coates1 Mullard Space Science Laboratory, UCL, UK
Atmospheric escape A.J.Coates1 Mullard Space Science Laboratory, UCL, UK With thanks to T.Cravens for some slides Atmospheric escape • Importance of escape to planetary evolution • Escape mechanisms – Thermal escape – Non-thermal mechanisms • Ion pickup • Bulk plasma escape along tail • Photoelecton-induced ambipolar escape • Measurements Importance of escapee • Mars has no global magnetic field • Estimated loss rate ~1025 (0.1-0.5 kg) s-1 (Lundin et al) – significant on solar system timescale High sputtering rates (Kass et al 96) Low Lammer et al 2002 sputtering rates (Luhmann et al 92) Thermal escape mechanism V (O) V (H) • Plot shows the speed 0 0 distributions for oxygen & hydrogen atoms in Earth’s exosphere, at a typical exosphere temperature T=1000 K • The most probable value is v0, which turns out to be 2kT v = 0 m • The fraction of oxygen atoms above the escape velocity (11.2 km s-1) is negligible, whereas a significant fraction of hydrogen atoms are above the escape velocity. • (As the high velocity atoms escape, the distribution quickly returns to this equilibrium shape, so that more atoms can escape.) Fig from Salby, Fundamentals of Atmospheric physics Thermal (Jeans) escape Given a Maxwellian distribution, the upward flux of escaping particles (per unit area) is given by the Jeans formula for escape by thermal evaporation: 2 2 n(z ) v 0 ⎛ v esc ⎞ ⎛ v esc ⎞ ⎜ 1⎟ exp⎜ ⎟ -2 -1 Φ ecape = ⎜ 2 + ⎟ ⎜ − 2 ⎟ particles m s 2 π ⎝ v 0 ⎠ ⎝ v 0 ⎠ where n(z) is the number density 2kT is the most probable velocity, as above v 0 = m 2GM planet v esc = is the escape velocity (R planet + z) – Important factor is the final exponential term. -
Polymorphism of Sulfur: Structural and Dynamical Aspects Laura Crapanzano
Polymorphism of sulfur: Structural and Dynamical Aspects Laura Crapanzano To cite this version: Laura Crapanzano. Polymorphism of sulfur: Structural and Dynamical Aspects. Physics [physics]. Université Joseph-Fourier - Grenoble I, 2006. English. tel-00204149 HAL Id: tel-00204149 https://tel.archives-ouvertes.fr/tel-00204149 Submitted on 14 Jan 2008 HAL is a multi-disciplinary open access L’archive ouverte pluridisciplinaire HAL, est archive for the deposit and dissemination of sci- destinée au dépôt et à la diffusion de documents entific research documents, whether they are pub- scientifiques de niveau recherche, publiés ou non, lished or not. The documents may come from émanant des établissements d’enseignement et de teaching and research institutions in France or recherche français ou étrangers, des laboratoires abroad, or from public or private research centers. publics ou privés. Th`ese pr´esent´eepar Laura Crapanzano Pour obtenir le titre de Docteur de l’Universit´eJoseph Fourier - Grenoble I Polymorphism of Sulfur: Structural and Dynamical Aspects Date de Soutenance: 20 Juin 2005 Composition du jury: Dr. Joel Chevrier President du jury Dr. Paul Loubeyre Rapporteur Prof. Ralf Steudel Rapporteur Prof. Daniele Fioretto Examinateur Prof. Alfredo Segura Examinateur Prof. Robert Bellissent Directeur de th`ese Dr. Giulio Monaco Co-Directeur de th`ese Dr. Wilson Crichton Co-Directeurs de th`ese: Dr. Mohamed Mezouar Th`esepr´epar´eeau sein du laboratoire European Synchrotron Radiation Facility BP 220 - 38043 Grenoble Cedex - France Dedicated to my parents iv Abstract This thesis deals with an investigation of polymorphism in both solid and liquid state of sulphur. Emphasis has been put on the polymer transition of liquid sulphur. -
On the Reactivity of Nanoparticulate Elemental Sulfur
ON THE REACTIVITY OF NANOPARTICULATE ELEMENTAL SULFUR: EXPERIMENTATION AND FIELD OBSERVATIONS Fotios Christos Kafantaris Submitted to the faculty of the University Graduate School in partial fulfillment of the requirements for the degree Doctor of Philosophy in the Department of Earth Sciences, Indiana University December 2017 ii Accepted by the Graduate Faculty of Indiana University, in partial fulfillment of the requirements for the degree of Doctor of Philosophy. Doctoral Committee ___________________________ Gregory K. Druschel, PhD, Chair ___________________________ Kevin Mandernack, PhD ___________________________ William P. Gilhooly III, PhD ___________________________ Gabriel Filippelli, PhD ___________________________ Steven E. Lacey, PhD October 2, 2017 ___________________________ Brandy M. Toner, PhD iii © 2017 Fotios Christos Kafantaris iv DEDICATION I would like to dedicate this work to three women. The first one is the Most Holy Theotokos and Ever-Virgin Mary, the most precious individual the human race has and will ever have, the Bridge from earth to Heaven and the Gate to Paradise. Through Her intercessions to the Holy Trinity I am still alive and safe. The second woman is my mother, Eleni, who is the angel-on-earth that protects, nourishes, teaches, provides, inspires and guides me in life. Words would be poor to attempt to describe her and her virtues in an accurate manner. My mother is the main contributor of what I have become so far in life. The third woman is my σύζυγος (spouse) Diana, who has given me life, as well as meaning for life. Diana is the main contributor of what I will hopefully do in life from this point onward, and through her help I will hopefully manage to be with the other two forever. -
Atmospheric Escape from Mars: Lessons for Studies of Exoplanets
Exoplanets in our Backyard 2020 (LPI Contrib. No. 2195) 3039.pdf Atmospheric Escape from Mars: Lessons for Studies of Exoplanets. D. Brain1, M. Chaffin1, S. Curry2, H. Egan1, R. Ramstad1, B. Jakosky1, J. Luhmann2, C. Dong3, and R. Yelle4, 1LASP / University of Colorado (da- [email protected]), 2SSL / University of California Berkeley, 3PPPL / Princeton University, 4LPL / Univer- sity of Arizona, 2SSL / University of California Berkeley. Introduction: The planet Mars provides an intri- accelerated by electric fields near Mars. In addition, guing laboratory for investigations of habitability on MAVEN’s data have been used to validate models for exoplanets. Though conditions at the Martian surface the loss of atmosphere from the planet. We consider today are not well-suited for life, the Martian surface how each of these ‘pathways’ for atmospheric loss and atmosphere hold many clues that suggest that the would be changed if: the stellar photon and particle necessary conditions for life were present billions of flux were consistent with a typical (such as it is) M years ago. Habitable surface conditions long ago are Dwarf star, stellar disturbed (i.e. storm) periods were thought to require a substantially different atmos- more frequent and more intense, and the planet orbit- phere, implying that much of the Martian atmosphere ed at a closer distance from the star. We examine the escaped to space over time. Mars may have been par- influence of these changes on thermal escape of hy- ticularly susceptible to escape (compared to Earth) drogen, photochemical escape of oxygen, ion loss, because of its small size and/or its lack of global and sputtering. -
Chemistry of Sulfur (Z=16) Learning Objectives Describe the Chemistry of the Oxygen Group
Chemistry of Sulfur (Z=16) Learning Objectives Describe the chemistry of the oxygen group. Give the trend of various properties. Remember the names of Group 16 elements. Explain the Frasch process. Describe properties and applications of H2SO4 . Explain properties and applications of H2S. Sulfur is a chemical element that is represented with the chemical symbol "S" and the atomic number 16 on the periodic table. Because it is 0.0384% of the Earth's crust, sulfur is the seventeenth most abundant element following strontium. Sulfur also takes on many forms, which include elemental sulfur, organo-sulfur compounds in oil and coal, H2S(g) in natural gas, and mineral sulfides and sulfates. This element is extracted by using the Frasch process (discussed below), a method where superheated water and compressed air is used to draw liquid sulfur to the surface. Offshore sites, Texas, and Louisiana are the primary sites that yield extensive amounts of elemental sulfur. However, elemental sulfur can also be produced by reducing H2S, commonly found in oil and natural gas. For the most part though, sulfur is used to produce SO2(g) and H2SO4. Known from ancient times (mentioned in the Hebrew scriptures as brimstone) sulfur was classified as an element in 1777 by Lavoisier. Pure sulfur is tasteless and odorless with a light yellow color. Samples of sulfur often encountered in the lab have a noticeable odor. Sulfur is the tenth most abundant element in the known universe. Sulfur at a Glance Atomic Number 16 Atomic Symbol S Atomic Weight 32.07 grams per mole Structure orthorhombic Phase at room temperature solid Classification nonmetal Physical Properties of Sulfur Sulfur has an atomic weight of 32.066 grams per mole and is part of group 16, the oxygen family.