Leucocratic & Gabbroic Xenoliths from Hualalai
Total Page:16
File Type:pdf, Size:1020Kb
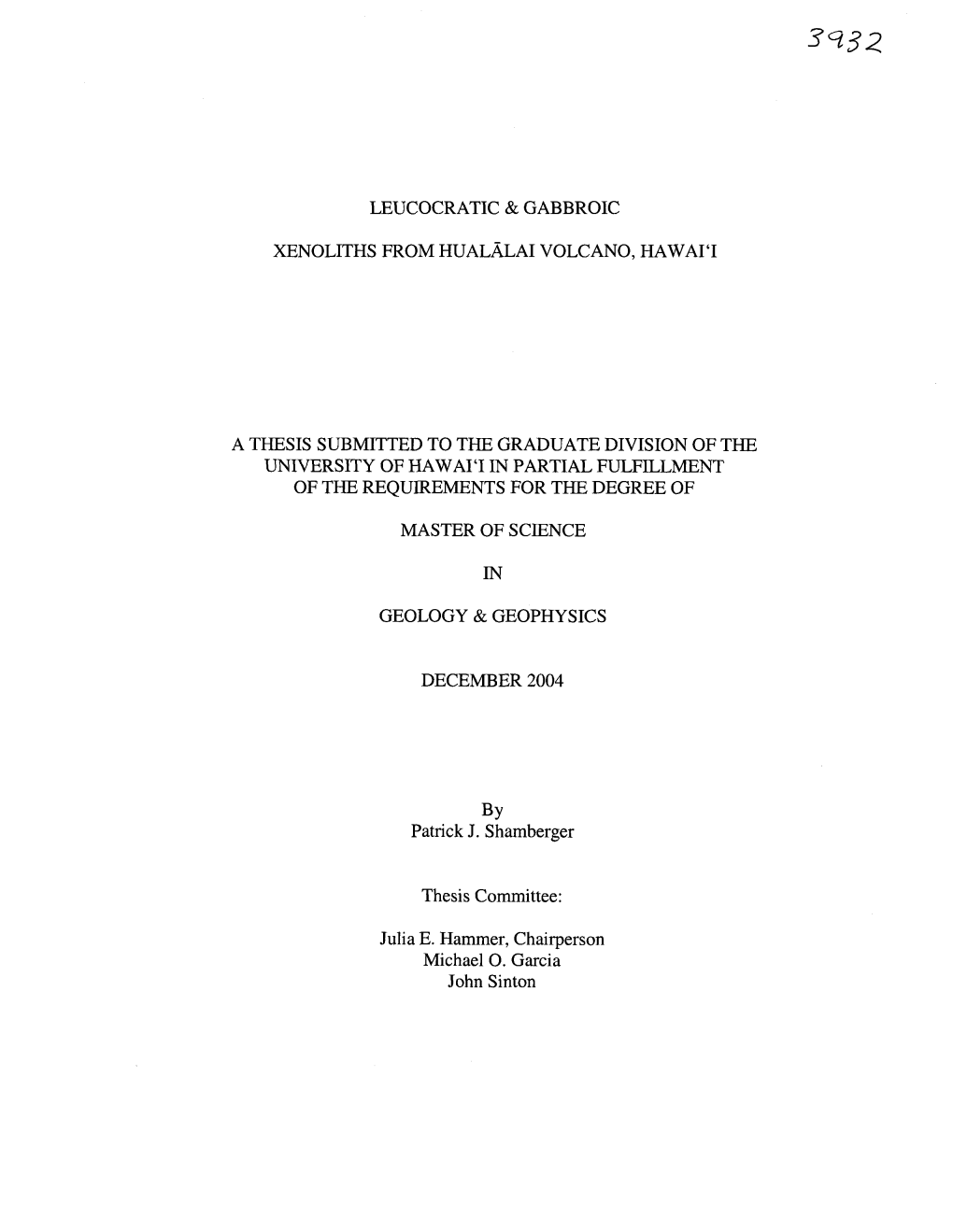
Load more
Recommended publications
-
Comments On'kaersutite from San Carlos, Arizona, with Comments On
MINERALOGICAL MAGAZINE, SEPTEMBER I969, VOL. 37, NO. 287 Comments on 'Kaersutite from San Carlos, Arizona, with comments on the paragenesis of this mineral' by Brian Mason MARTIN PRINZ ~ Department of Geology, University of New Mexico, Albuquerque, New Mexico 87Io6 AND C. E. NEHRU Department of Geology, Brooklyn College, Brooklyn, New York II2~O s u MM A R Y. Electron probe analyses have been made of eight kaersutites from San Carlos, A~cizona, and one from Kakanui, New Zealand; also of olivine, clinopyroxene, ilmenite, and spinel from San Carlos. There is not as yet adequate evidence that the kaersutite is an upper mantle phase. MASON (I968a) suggests that kaersutite, as exemplified by the occurrence at San Carlos, Arizona, as well as from some other localities, may represent the amphibole phase in upper mantle rocks. The possibility of amphibole as an upper mantle phase was most clearly suggested by Oxburgh (I964) since it would help explain the alkali content of basaltic rocks, which otherwise would be derived from alkali-poor ultra- mafic mantle rocks. Since we are carrying out a detailed study of the ultramafic inclusions of the San Carlos, Arizona, locality, we would like to clarify some of the petrologic significance of the kaersutite from this area because it leads us to different conclusions from those of Mason. Hopefully, our comments will be of value to others suggesting amphibole as an upper mantle phase. San Carlos locality. The kaersutite described by Mason (I968a) in the San Carlos locality occurs as a granular aggregate of anhedral crystals, with a little inter-granular ilmenite. -
Kaersutite-Bearing Xenoliths and Megacrysts in Volcanic Rocks from the Funk Seamount in the Southwest Indian Ocean
Kaersutite-bearing xenoliths and megacrysts in volcanic rocks from the Funk Seamount in the southwest Indian Ocean ARCH M. REID* AND ANTON P. LE ROEX Department of Geology, University of Cape Town, Rondebosch 7700, South Africa Abstract Eight samples (seven volcanic rocks and one quartz sandstone) have been dredged from the Funk Seamount, 60 km NW of Marion Island in the southwest Indian Ocean Oat. 46 ~ 15' S, long. 37 ~ 20' E). The volcanic rocks are fine-grained vesicular basanitoids and glass-rich volcanic breccias geochemically similar to the Marion Island lavas. Lavas and breccias contain a suite of megacryst minerals and of small polymineralic xenoliths, in both of which kaersutite is a prominent constituent. The megacryst suite comprises large unzoned single grains of kaersutite, plagioclase, pyroxene, magnetite and ilmenite, all showing textural evidence of resorption/reaction with the basanitoid host. The megacrysts have a limited range of compositions except for the plagioclase which ranges from oligoclase to labradorite. The small (2 mm to ~ 3 cm) xenoliths are mostly two-pyroxene amphibole assemblages with or without olivine, magnetite, ilmenite, plagioclase and apatite. The xenoliths show some evidence of reaction with the basanitoid host and most have undergone recrystallization and/or localised decompression melting. Xenolith and megacryst assemblages are interpreted as being associated with the formation and partial crystallization of a hydrous basanitoid melt at depth. KEYWORDS: kaersutite, amphibole, xenolith, Funk Seamount, Indian Ocean. Introduction megacrysts. Brown amphibole is a prominent con- stituent of both xenolith and megacryst suites. F U N K S E A M O U N T is a prominent seamount situa- Mantle xenoliths in abyssal lavas are rare and so ted 60 km to the NW of Marion and Prince Edward these samples are of particular interest. -
Amphibole–Melt Disequilibrium in Silicic Melt of the Aso-4 Caldera
Ishibashi et al. Earth, Planets and Space (2018) 70:137 https://doi.org/10.1186/s40623-018-0907-4 FULL PAPER Open Access Amphibole–melt disequilibrium in silicic melt of the Aso‑4 caldera‑forming eruption at Aso Volcano, SW Japan Hidemi Ishibashi1*, Yukiko Suwa1, Masaya Miyoshi2, Atsushi Yasuda3 and Natsumi Hokanishi3 Abstract The most recent and largest caldera-forming eruption occurred at ~ 90 ka at Aso Volcano, SW Japan, and is known as the “Aso-4 eruption.” We performed chemical analyses of amphibole phenocrysts from Aso-4 pyroclasts collected from the initial and largest pyroclastic unit (4I-1) of the eruption to infer the composition–temperature–pressure conditions of the melt that crystallized amphibole phenocrysts. Each amphibole phenocryst is largely chemically homogeneous, but inter-grain chemical variation is observed. Geothermometry, geobarometry, and melt–SiO2 relationships based on amphibole single-phase compositions reveal that most amphibole phenocrysts were in equilibrium with hydrous SiOmelt melt comprising ~ 63–69 wt% SiO2 ( 2 ) at 910–950 °C, although several grains were crystallized from more mafc and higher-temperature melts (~ 57–60.5 wt% SiO2 and 965–980 °C). The amphibole temperatures are comparable with those previously estimated from two-pyroxene geothermometry, but are much higher than temperatures previ- melt ously estimated from Fe–Ti oxide geothermometry. The estimated SiO2 contents are lower than that of the host melt in the 4I-1 pyroclasts. Chemical and thermal disequilibrium between the amphibole rims and the host melt, as well as intra-grain homogeneity and inter-grain heterogeneity of amphibole compositions, suggests that these amphiboles were incorporated into the host melt immediately prior to the caldera-forming eruption. -
New Minerals Approved Bythe Ima Commission on New
NEW MINERALS APPROVED BY THE IMA COMMISSION ON NEW MINERALS AND MINERAL NAMES ALLABOGDANITE, (Fe,Ni)l Allabogdanite, a mineral dimorphous with barringerite, was discovered in the Onello iron meteorite (Ni-rich ataxite) found in 1997 in the alluvium of the Bol'shoy Dolguchan River, a tributary of the Onello River, Aldan River basin, South Yakutia (Republic of Sakha- Yakutia), Russia. The mineral occurs as light straw-yellow, with strong metallic luster, lamellar crystals up to 0.0 I x 0.1 x 0.4 rnrn, typically twinned, in plessite. Associated minerals are nickel phosphide, schreibersite, awaruite and graphite (Britvin e.a., 2002b). Name: in honour of Alia Nikolaevna BOG DAN OVA (1947-2004), Russian crys- tallographer, for her contribution to the study of new minerals; Geological Institute of Kola Science Center of Russian Academy of Sciences, Apatity. fMA No.: 2000-038. TS: PU 1/18632. ALLOCHALCOSELITE, Cu+Cu~+PbOZ(Se03)P5 Allochalcoselite was found in the fumarole products of the Second cinder cone, Northern Breakthrought of the Tolbachik Main Fracture Eruption (1975-1976), Tolbachik Volcano, Kamchatka, Russia. It occurs as transparent dark brown pris- matic crystals up to 0.1 mm long. Associated minerals are cotunnite, sofiite, ilin- skite, georgbokiite and burn site (Vergasova e.a., 2005). Name: for the chemical composition: presence of selenium and different oxidation states of copper, from the Greek aA.Ao~(different) and xaAxo~ (copper). fMA No.: 2004-025. TS: no reliable information. ALSAKHAROVITE-Zn, NaSrKZn(Ti,Nb)JSi401ZJz(0,OH)4·7HzO photo 1 Labuntsovite group Alsakharovite-Zn was discovered in the Pegmatite #45, Lepkhe-Nel'm MI. -
Petrology of Inclusion-Rich Lavas at Minna Bluff, Mcmurdo Sound, Antarctica: Implications for Magma Origin, Differentiation, and Eruption Dynamics
PETROLOGY OF INCLUSION-RICH LAVAS AT MINNA BLUFF, MCMURDO SOUND, ANTARCTICA: IMPLICATIONS FOR MAGMA ORIGIN, DIFFERENTIATION, AND ERUPTION DYNAMICS Mary K. Scanlan A Thesis Submitted to the Graduate College of Bowling Green State University in partial fulfillment of the requirements for the degree of MASTER OF SCIENCE August 2008 Committee: Kurt Panter (Ph.D.), Advisor John Farver (Ph.D.) Thom Wilch (Ph.D.) © 2008 Mary Scanlan All Rights Reserved iii ABSTRACT Kurt Panter, Advisor Xeno Ridge, a newly discovered group of inclusion-rich deposits located at the top of the Minna Bluff stratigraphic section, formed as a result of magmatic mixing/mingling and provides insight into magma origins, mixing and eruption dynamics, and the evolution of Minna Bluff. Phonolite to tephriphonolite lavas are contain abundant inclusions which vary in size, shape, and mineralogy. Five inclusion types are identified at Xeno Ridge. The lavas that host the inclusions are dark-gray and porphyritic with a hypocrystalline, vesicular groundmass. Minerals within the host lavas include feldspar (plagioclase = An27–An84, alkali feldspar = Ab41Or57−Ab70Or20), amphibole (kaersutite), diopside (~Wo55En35Fs10), titanomagnetite (Ti# 55), olivine (Fo45, Fo81), and apatite. Type I inclusions are highly vesicular with sinuous forms and crenulate margins with the host indicating magmatic mixing/mingling. The mineral assemblage of Type I includes kaersutite (15-20 vol. %), feldspar (plagioclase = An33−An80, alkali feldspar = ~Ab55Or45), diopside (~Wo55En30Fs6), titanomagnetite (Ti# ~75), olivine (Fo48, Fo85) and apatite. Type II inclusions are kaersutite megacrysts and glomerocrysts dominated by kaersutite with subordinate phenocrysts, microphenocrysts and groundmass composed of plagioclase (An20 – An76), titanomagnetite (Ti# 84), diopside (~Wo50En35Fs15), and apatite. Type III is a single porphyritic inclusion with phenocrysts of anorthoclase and is similar in texture and mineralogy to lavas found within the Minna Bluff stratigraphic section. -
List of Abbreviations
List of Abbreviations Ab albite Cbz chabazite Fa fayalite Acm acmite Cc chalcocite Fac ferroactinolite Act actinolite Ccl chrysocolla Fcp ferrocarpholite Adr andradite Ccn cancrinite Fed ferroedenite Agt aegirine-augite Ccp chalcopyrite Flt fluorite Ak akermanite Cel celadonite Fo forsterite Alm almandine Cen clinoenstatite Fpa ferropargasite Aln allanite Cfs clinoferrosilite Fs ferrosilite ( ortho) Als aluminosilicate Chl chlorite Fst fassite Am amphibole Chn chondrodite Fts ferrotscher- An anorthite Chr chromite makite And andalusite Chu clinohumite Gbs gibbsite Anh anhydrite Cld chloritoid Ged gedrite Ank ankerite Cls celestite Gh gehlenite Anl analcite Cp carpholite Gln glaucophane Ann annite Cpx Ca clinopyroxene Glt glauconite Ant anatase Crd cordierite Gn galena Ap apatite ern carnegieite Gp gypsum Apo apophyllite Crn corundum Gr graphite Apy arsenopyrite Crs cristroballite Grs grossular Arf arfvedsonite Cs coesite Grt garnet Arg aragonite Cst cassiterite Gru grunerite Atg antigorite Ctl chrysotile Gt goethite Ath anthophyllite Cum cummingtonite Hbl hornblende Aug augite Cv covellite He hercynite Ax axinite Czo clinozoisite Hd hedenbergite Bhm boehmite Dg diginite Hem hematite Bn bornite Di diopside Hl halite Brc brucite Dia diamond Hs hastingsite Brk brookite Dol dolomite Hu humite Brl beryl Drv dravite Hul heulandite Brt barite Dsp diaspore Hyn haiiyne Bst bustamite Eck eckermannite Ill illite Bt biotite Ed edenite Ilm ilmenite Cal calcite Elb elbaite Jd jadeite Cam Ca clinoamphi- En enstatite ( ortho) Jh johannsenite bole Ep epidote -
Durham Research Online
Durham Research Online Deposited in DRO: 08 February 2019 Version of attached le: Accepted Version Peer-review status of attached le: Peer-reviewed Citation for published item: Humphreys, Madeleine C. S. and Cooper, George F. and Zhang, Jing and Loewen, Matthew and Kent, Adam J. R. and Macpherson, Colin G. and Davidson, Jon P. (2019) 'Unravelling the complexity of magma plumbing at Mount St. Helens : a new trace element partitioning scheme for amphibole.', Contributions to mineralogy and petrology., 174 (1). p. 9. Further information on publisher's website: https://doi.org/10.1007/s00410-018-1543-5 Publisher's copyright statement: This is a post-peer-review, pre-copyedit version of an article published in Contributions to mineralogy and petrology. The nal authenticated version is available online at: https://doi.org/10.1007/s00410-018-1543-5 Additional information: Use policy The full-text may be used and/or reproduced, and given to third parties in any format or medium, without prior permission or charge, for personal research or study, educational, or not-for-prot purposes provided that: • a full bibliographic reference is made to the original source • a link is made to the metadata record in DRO • the full-text is not changed in any way The full-text must not be sold in any format or medium without the formal permission of the copyright holders. Please consult the full DRO policy for further details. Durham University Library, Stockton Road, Durham DH1 3LY, United Kingdom Tel : +44 (0)191 334 3042 | Fax : +44 (0)191 334 2971 https://dro.dur.ac.uk 1 Unravelling the complexity of magma plumbing at Mount St Helens: a new trace element 2 partitioning scheme for amphibole 3 4 Madeleine. -
Mineralogy Lab Manual 4-9.Pdf
Rock forming minerals The Mineral Olivine ! • Chemistry: (Mg, Fe)2SiO4, Magnesium Iron Silicate. • Class: Silicates • Subclass: Nesosilicates • Group: Olivine • Uses: As gemstones, industrial uses as refractory sands and abrasives, an ore of magnesium and as mineral specimens. • Group: Olivine is actually a name for a series between two end members, fayalite and forsterite. Fayalite is the iron rich member with a pure formula of Fe2SiO4. • Forsterite is the magnesium rich member with a pure formula of Mg2SiO4. • the series includes: - Ca2SiO4 - larnite ! - Mn2SiO4 - tephroite! ! - CaMgSiO4 - monticellite (which is commonly found in metamorphosed dolomites) ! Physical properties for hand sample • Color is a light near emerald green to the more common pale yellowish green; also found colorless, greenish brown to black. • Luster is vitreous. • Transparency: Crystals are transparent to translucent. • Crystal System is orthorhombic; • Habits include flatten tabular to box shaped crystals, but good crystals are rare. More commonly found as grains in alluvial gravels and as granular xenoliths in magnesium rich volcanic rock. Also massive. Twinning is rare, but has produced star shaped trillings. • Cleavage is poor in two directions at 90 degrees, is more distinct in fayalite. • Fracture is conchoidal. • Hardness is 6.5 - 7. • Specific Gravity is approximately 3.2 for forsterite - 4.3 for fayalite (above average for non-metallic minerals). • Streak is white. • Associated Minerals are pyroxene group minerals, ca-felspars, amphiboles. • Common rocks: ultramafic, mafic rocks (Dunite, peridotite, basalt, gabbro). It is also found in metamorphic rocks and Serpentine deposits as a primary mineral. Olivine may also occur in meteorites. • It weathers to iddingsite (a combination of clay minerals, iron oxides and ferrihydrites) ! readily in the presence of water. -
Cl-Rich Amphiboles As Record for Hydrothermal Processes at Very
Cl-rich amphiboles as record for hydrothermal processes at very high temperatures in the deep oceanic crust: brine/rock interaction experiments and investigation on natural rocks Von der Naturwissenschaftlichen Fakultät der Gottfried Wilhelm Leibniz Universität Hannover zur Erlangung des Grades Doktorin der Naturwissenschaften (Dr. rer. nat.) genehmigte Dissertation von Adriana Miriam Currin Sala, M. Sc (Niederlande) 2018 Referent: Prof. Dr. rer. nat. Jürgen Koepke Korreferenten: Prof. Dr. rer. nat. Wolfgang Bach Prof. Dr. sci. nat. Ulrich Heimhofer Tag der Promotion: 15.11.2018 2 Abstract Interactions between rock and high temperature seawater-derived fluids are recorded in hydrothermal veins and dykelets that cross-cut layered olivine gabbros deep in the plutonic section of the Samail Ophiolite, Wadi Wariyah, Sultanate of Oman. Here we present a study – using petrographic, microanalytical, isotopic, and structural methods – of amphiboles found in the aforementioned veins and dykelets, which show a conspicuous compositional variation from high-Ti magnesiohastingsite and pargasite via magnesiohornblende and edenite, to Cl-rich ferropargasite and hastingsite (with up to 5.4 wt% Cl) and actinolite. These minerals record a wide range of formation conditions from magmatic to hydrothermal at varying water/rock ratios and salinities, while the formation of super Cl-rich amphibole suggests the occurrence of phase separation, and 87Sr/86Sr and stable δ18O isotope analyses confirm the influence of a hydrothermal fluid in a rock-dominated environment. A parallel experimental study was conducted at hydrothermal (500 – 750 °C) and magmatic (900 °C) conditions at pressures of 2 kbar, and fO2 close to NNO, with an amphibole-containing natural olivine gabbro and saline fluid (6, 20 and 50 wt% NaCl). -
Upper Mantle and Crustal Origin of Olivine-Pyroxene-Kaersutite
VllA-12 0830h POSTER Upper Mantle and Crustal Origin of Olivine-Pyroxene- Kaersutite Megacryats, and Peridotite Curnulates and Xenoliths Rom the Bandama Volcanic Complex (Gran Ca- naria, Spain) Jose Mangas1 (36928451296; joae.mangasQhsica.ulpgc.es) Robert Clac&iatti2 (33-1-69084815; cloc&iatti@drecam cedr) Ftancisco Jase Perez-Torrado' (34-928451298; francisco.perezt3 fisi~a.ulpgces) Dominique Massare2 (33-1-69084815) (Sponsor: Emilio Herrer+A~rvpr~! 'Departamento de Fisica, Campup de Tafira, ULPGC, Las Palmas 35.017. Spain 2Laboratorie Pierre Sue, CEA, Gif sur lvette 91191, hance The Quatcrnary volcamc complex of Bandama is formed by a strornbo- lian cone and a caldera of phreatomagmatic-collapse origin It shows two lava flows of basanite cornposition and fall and base surge pyro- clastic deposits The lava Rows have alivine and augstediapside phe- riocrysts and as subordinate minerals spinel and magnetite. The pyro- ilastic deposits shvw olivine. clinopyroxene and kaersutite phenocrysts and megacrysts, pendotite cumulates (dimite, wehrlite, clinopyroxen- ite wtth olivinp, rhnopirmenite) and xenoliths (dunite 2nd Iherzolite) containing olivinc, augite-diopstde, enslatite, spmel, magnetite, phlo- gopite, apatite and pyrrhotitepentlandite Taking into aceount the val- canologd study and the microscopic, electron microprobe, microther- mometric (fluid and melt inclusions) and bulk-rock analysis of the 26 samples. we concliide that the mincrals which make up the megacrysts, curnulates and xenoliths dsplay dlfTerent characteristm between them and from the ratio to minerals oí the lava flow We interprete that thme samples were original4 under difieren1 LhermobarogeochemiraI conditions and that the basanitic magma which gave rise to the Ban- dama complex ascended fram upper mantle to the surface (-33 km ta O 5 km), trapping pendotile ciirnulates and xenoliths, and megacrysts of diKerents depths and genesis Irom the spinel stability field in the uppcr mantle lo the owanir crust (-27 km to -4.5 km) . -
Magnesio-Hastingsite Naca2[(Mg; Fe )4Fe ](Si6al2)O22(OH)2 C 2001 Mineral Data Publishing, Version 1.2 ° Crystal Data: Monoclinic
2+ 3+ Magnesio-hastingsite NaCa2[(Mg; Fe )4Fe ](Si6Al2)O22(OH)2 c 2001 Mineral Data Publishing, version 1.2 ° Crystal Data: Monoclinic. Point Group: 2=m: [Prismatic.] Twinning: [Simple or multiple twinning 100 .] k f g Physical Properties: Cleavage: [Perfect on 110 , with intersections at 56± and 124±; partings on 001 , 100 .] Tenacity: [Brittle.] fHardgness = [5{6] D(meas.») = 3.18{3».22 f g f g D(calc.) = 3.243 Optical Properties: Semitransparent. Color: Green. Luster: [Vitreous.] Optical Class: Biaxial ({). Pleochroism: X = pale brown; Y = dark brown; Z = green-brown. Orientation: Y = b; Z c 15 {19 . ® = 1.652{1.676 ¯ = 1.664{1.687 ° = 1.672{1.695 ^ ' ± ± 2V(meas.) = 80±{84± Cell Data: Space Group: C2=m: a = 9.880(2) b = 18.012(4) c = 5.324(2) ¯ = 105:26(2)± Z = 2 X-ray Powder Pattern: n.d. Chemistry: (1) (2) (1) (2) SiO2 40.26 39.83 MgO 13.98 14.44 TiO2 3.16 2.56 CaO 12.01 12.39 Al2O3 11.99 14.98 Na2O 2.54 2.27 Fe2O3 7.78 7.66 K2O 1.87 1.25 + FeO 5.52 3.78 H2O 1.63 0.58 MnO 0.12 0.00 Total 100.86 99.74 3+ 2+ (1) Unteriefenbach, Austria; corresponds to (Ca1:90Na0:73K0:35)§=2:98(Mg3:07Fe0:86Fe0:68Ti0:35 Mn0:02Al0:02)§=5:00(Si5:94Al2:06)§=8:00O22(OH)1:60: (2) B·rezno nad Labem (Grosspriesen), 3+ 2+ Czech Republic; corresponds to (Ca1:97Na0:65K0:24)§=2:86(Mg3:20Fe0:86Al0:54Fe0:47Ti0:29)§=5:36 (Si5:92Al2:08)§=8:00O22(OH)0:58: Polymorphism & Series: Forms a series with hastingsite. -
Rock-Forming Minerals of Lamprophyres and Associated Mafic Dykes from the KruNé Hory/Erzgebirge (Czech Republic)
Journal of the Czech Geological Society 47/12(2002) 23 Rock-forming minerals of lamprophyres and associated mafic dykes from the Kruné hory/Erzgebirge (Czech Republic) Horninotvorné minerály lamprofyrù a pøíbuzných mafických ilných hornin z Kruných hor (Èeská republika) (4 figs, 5 tabs) EDVÍN PIVEC1 FRANTIEK V. HOLUB2 MILO LANG1 JIØÍ K. NOVÁK1 MIROSLAV TEMPROK2 1 Geological Institute, Academy of Sciences of the Czech Republic, Rozvojová 135, CZ 16502 Praha 6, Czech Republic 2 Charles University, Faculty of Science, Albertov 6, CZ 12843 Praha 2, Czech Republic; e-mail: [email protected], [email protected] Electron microprobe analyses were made on micas, amphiboles, feldspars, chlorites and accessory minerals in lamprophyric dykes (kersantites, minettes, spessartites), and in associated mafic diorite to tonalite porphyries (porphyrites) of the Kruné hory (Erzgebirge) area and Mariánské Láznì region in Western Bohemia (Czech Republic). All studied rocks are altered to various degrees during deuteric and/or postmagmatic stages of evolution. The only primary mafic mineral preserved in all rock types is Mg-biotite to phlogopite, in spessartites and some diorite porphyries also Ti-rich hornblende corresponding to titanian magnesiohastingsite to kaersutite. Magmatic biotites are relatively rich in Ti with limited variations in their Mg/Fe ratios, evidently re-equilibrated during cooling or re-heating. Olivine is totally replaced by pilitic pseudomorphs and by biotite-actinolite clots. Phenocrystic clinopyroxene is completely uralitized, often in well-preserved original shapes. The secondary amphiboles correspond to Si-rich magnesiohornblende to actinolite. Chlorite and epidote are rather scarce and their impor- tant occurrences are restricted to limited number of samples.