Cataclysmic Variables: Areview
Total Page:16
File Type:pdf, Size:1020Kb
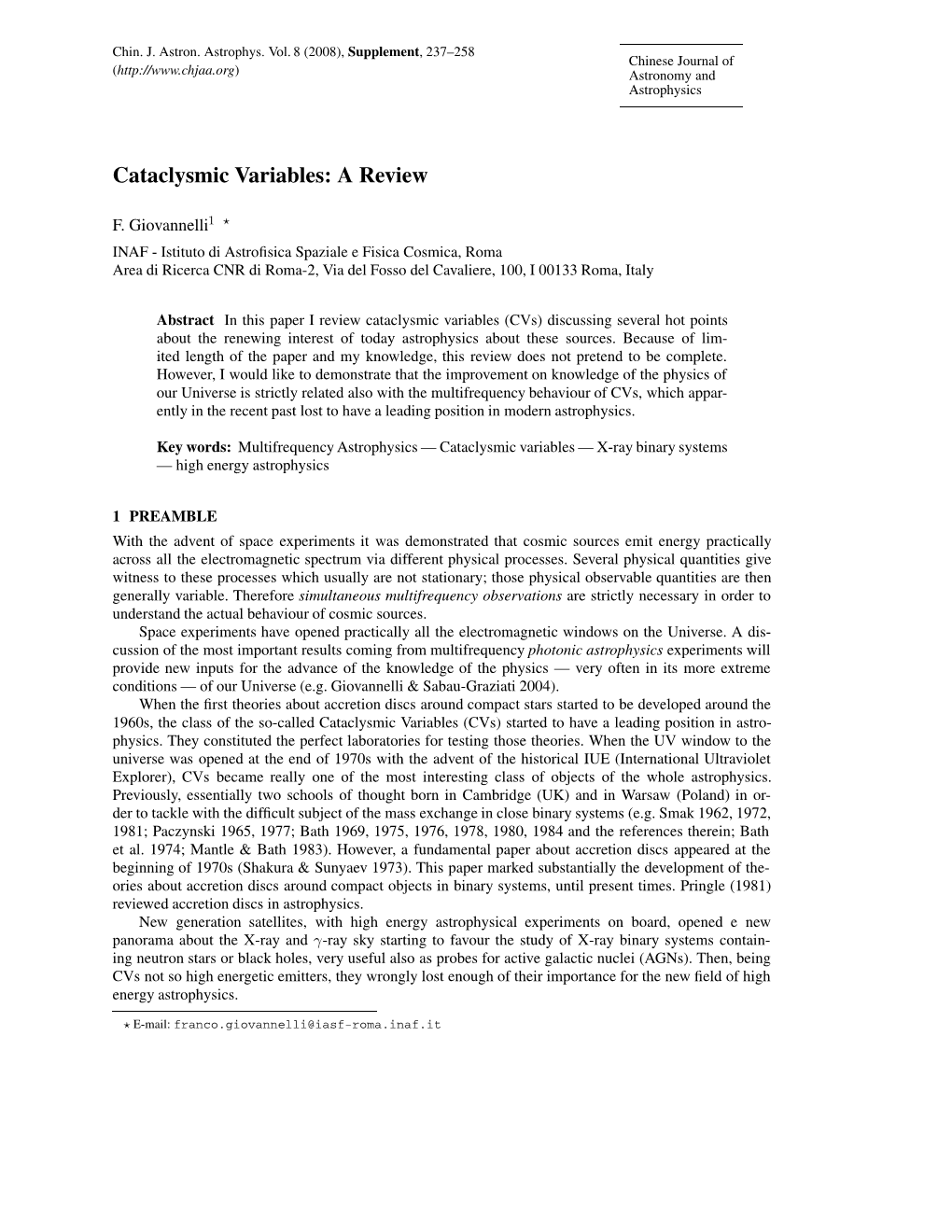
Load more
Recommended publications
-
White Dwarfs and Electron Degeneracy
White Dwarfs and Electron Degeneracy Farley V. Ferrante Southern Methodist University Sirius A and B 27 March 2017 SMU PHYSICS 1 Outline • Stellar astrophysics • White dwarfs • Dwarf novae • Classical novae • Supernovae • Neutron stars 27 March 2017 SMU PHYSICS 2 27 March 2017 SMU PHYSICS 3 Pogson’s ratio: 5 100≈ 2.512 27 March 2017 M.S. Physics Thesis Presentation 4 Distance Modulus mM−=5 log10 ( d) − 1 • Absolute magnitude (M) • Apparent magnitude of an object at a standard luminosity distance of exactly 10.0 parsecs (~32.6 ly) from the observer on Earth • Allows true luminosity of astronomical objects to be compared without regard to their distances • Unit: parsec (pc) • Distance at which 1 AU subtends an angle of 1″ • 1 AU = 149 597 870 700 m (≈1.50 x 108 km) • 1 pc ≈ 3.26 ly • 1 pc ≈ 206 265 AU 27 March 2017 SMU PHYSICS 5 Stellar Astrophysics • Stefan-Boltzmann Law: 54 2π k − − −− FT=σσ4; = = 5.67x 10 5 ergs 1 cm 24 K bol 15ch23 • Effective temperature of a star: Temp. of a black body with the same luminosity per surface area • Stars can be treated as black body radiators to a good approximation • Effective surface temperature can be obtained from the B-V color index with the Ballesteros equation: 11 T = 4600+ 0.92(BV−+) 1.70 0.92(BV −+) 0.62 • Luminosity: 24 L= 4πσ rT* E 27 March 2017 SMU PHYSICS 6 H-R Diagram 27 March 2017 SMU PHYSICS 8 27 March 2017 SMU PHYSICS 9 White dwarf • Core of solar mass star • Pauli exclusion principle: Electron degeneracy • Degenerate Fermi gas of oxygen and carbon • 1 teaspoon would weigh 5 tons • No energy produced from fusion or gravitational contraction Hot white dwarf NGC 2440. -
Unknown Amorphous Carbon II. LRS SPECTRA the Sample Consists Of
Table I A summary of the spectral -features observed in the LRS spectra of the three groups o-f carbon stars. The de-finition o-f the groups is given in the text. wavelength Xmax identification Group I B - 12 urn E1 9.7 M™ Silicate 12 - 23 jim E IB ^m Silicate Group II < 8.5 M"i A C2H2 CS? 12 - 16 f-i/n A 13.7 - 14 Mm C2H2 HCN? 8 - 10 Mm E 8.6 M"i Unknown 10 - 13 Mm E 11.3 - 11 .7 M«> SiC Group III 10 - 13 MJn E 11.3 - 11 .7 tun SiC B - 23 Htn C Amorphous carbon 1 The letter in this column indicates the nature o-f the -feature: A = absorption; E = emission; C indicates the presence of continuum opacity. II. LRS SPECTRA The sample consists of 304 carbon stars with entries in the LRS catalog (Papers I-III). The LRS spectra have been divided into three groups. Group I consists of nine stars with 9.7 and 18 tun silicate features in their LRS spectra pointing to oxygen-rich dust in the circumstellar shell. These sources are discussed in Paper I. The remaining stars all have spectra with carbon-rich dust features. Using NIR photometry we have shown that in the group II spectra the stellar photosphere is the dominant continuum. The NIR color temperature is of the order of 25OO K. Paper II contains a discussion of sources with this class of spectra. The continuum in the group III spectra is probably due to amorphous carbon dust. -
The Inter-Eruption Timescale of Classical Novae from Expansion of the Z Camelopardalis Shell
The Astrophysical Journal, 756:107 (6pp), 2012 September 10 doi:10.1088/0004-637X/756/2/107 C 2012. The American Astronomical Society. All rights reserved. Printed in the U.S.A. THE INTER-ERUPTION TIMESCALE OF CLASSICAL NOVAE FROM EXPANSION OF THE Z CAMELOPARDALIS SHELL Michael M. Shara1,4, Trisha Mizusawa1, David Zurek1,4, Christopher D. Martin2, James D. Neill2, and Mark Seibert3 1 Department of Astrophysics, American Museum of Natural History, Central Park West at 79th Street, New York, NY 10024-5192, USA 2 Department of Physics, Math and Astronomy, California Institute of Technology, 1200 East California Boulevard, Mail Code 405-47, Pasadena, CA 91125, USA 3 Observatories of the Carnegie Institution of Washington, 813 Santa Barbara Street, Pasadena, CA 91101, USA Received 2012 May 14; accepted 2012 July 9; published 2012 August 21 ABSTRACT The dwarf nova Z Camelopardalis is surrounded by the largest known classical nova shell. This shell demonstrates that at least some dwarf novae must have undergone classical nova eruptions in the past, and that at least some classical novae become dwarf novae long after their nova thermonuclear outbursts. The current size of the shell, its known distance, and the largest observed nova ejection velocity set a lower limit to the time since Z Cam’s last outburst of 220 years. The radius of the brightest part of Z Cam’s shell is currently ∼880 arcsec. No expansion of the radius of the brightest part of the ejecta was detected, with an upper limit of 0.17 arcsec yr−1. This suggests that the last Z Cam eruption occurred 5000 years ago. -
Information Bulletin on Variable Stars
COMMISSIONS AND OF THE I A U INFORMATION BULLETIN ON VARIABLE STARS Nos November July EDITORS L SZABADOS K OLAH TECHNICAL EDITOR A HOLL TYPESETTING K ORI ADMINISTRATION Zs KOVARI EDITORIAL BOARD L A BALONA M BREGER E BUDDING M deGROOT E GUINAN D S HALL P HARMANEC M JERZYKIEWICZ K C LEUNG M RODONO N N SAMUS J SMAK C STERKEN Chair H BUDAPEST XI I Box HUNGARY URL httpwwwkonkolyhuIBVSIBVShtml HU ISSN COPYRIGHT NOTICE IBVS is published on b ehalf of the th and nd Commissions of the IAU by the Konkoly Observatory Budap est Hungary Individual issues could b e downloaded for scientic and educational purp oses free of charge Bibliographic information of the recent issues could b e entered to indexing sys tems No IBVS issues may b e stored in a public retrieval system in any form or by any means electronic or otherwise without the prior written p ermission of the publishers Prior written p ermission of the publishers is required for entering IBVS issues to an electronic indexing or bibliographic system to o CONTENTS C STERKEN A JONES B VOS I ZEGELAAR AM van GENDEREN M de GROOT On the Cyclicity of the S Dor Phases in AG Carinae ::::::::::::::::::::::::::::::::::::::::::::::::::: : J BOROVICKA L SAROUNOVA The Period and Lightcurve of NSV ::::::::::::::::::::::::::::::::::::::::::::::::::: :::::::::::::: W LILLER AF JONES A New Very Long Period Variable Star in Norma ::::::::::::::::::::::::::::::::::::::::::::::::::: :::::::::::::::: EA KARITSKAYA VP GORANSKIJ Unusual Fading of V Cygni Cyg X in Early November ::::::::::::::::::::::::::::::::::::::: -
The Planets for 1955 24 Eclipses, 1955 ------29 the Sky and Astronomical Phenomena Month by Month - - 30 Phenomena of Jupiter’S S a Te Llite S
THE OBSERVER’S HANDBOOK FOR 1955 PUBLISHED BY The Royal Astronomical Society of Canada C. A. C H A N T, E d ito r RUTH J. NORTHCOTT, A s s is t a n t E d ito r DAVID DUNLAP OBSERVATORY FORTY-SEVENTH YEAR OF PUBLICATION P r i c e 50 C e n t s TORONTO 13 Ross S t r e e t Printed for th e Society By the University of Toronto Press THE ROYAL ASTRONOMICAL SOCIETY OF CANADA The Society was incorporated in 1890 as The Astronomical and Physical Society of Toronto, assuming its present name in 1903. For many years the Toronto organization existed alone, but now the Society is national in extent, having active Centres in Montreal and Quebec, P.Q.; Ottawa, Toronto, Hamilton, London, and Windsor, Ontario; Winnipeg, Man.; Saskatoon, Sask.; Edmonton, Alta.; Vancouver and Victoria, B.C. As well as nearly 1000 members of these Canadian Centres, there are nearly 400 members not attached to any Centre, mostly resident in other nations, while some 200 additional institutions or persons are on the regular mailing list of our publications. The Society publishes a bi-monthly J o u r n a l and a yearly O b s e r v e r ’s H a n d b o o k . Single copies of the J o u r n a l are 50 cents, and of the H a n d b o o k , 50 cents. Membership is open to anyone interested in astronomy. Annual dues, $3.00; life membership, $40.00. -
AT Cnc: a SECOND DWARF NOVA with a CLASSICAL NOVA SHELL
The Astrophysical Journal, 758:121 (5pp), 2012 October 20 doi:10.1088/0004-637X/758/2/121 C 2012. The American Astronomical Society. All rights reserved. Printed in the U.S.A. AT Cnc: A SECOND DWARF NOVA WITH A CLASSICAL NOVA SHELL Michael M. Shara1,5, Trisha Mizusawa1, Peter Wehinger2, David Zurek1,5,6, Christopher D. Martin3, James D. Neill3, Karl Forster3, and Mark Seibert4 1 Department of Astrophysics, American Museum of Natural History, Central Park West at 79th Street, New York, NY 10024-5192, USA 2 Steward Observatory, the University of Arizona, 933 North Cherry Avenue, Tucson, AZ 85721, USA 3 Department of Physics, Math and Astronomy, California Institute of Technology, 1200 East California Boulevard, Mail Code 405-47, Pasadena, CA 91125, USA 4 Observatories of the Carnegie Institution of Washington, 813 Santa Barbara Street, Pasadena, CA 91101, USA Received 2012 July 31; accepted 2012 August 23; published 2012 October 8 ABSTRACT We are systematically surveying all known and suspected Z Cam-type dwarf novae for classical nova shells. This survey is motivated by the discovery of the largest known classical nova shell, which surrounds the archetypal dwarf nova Z Camelopardalis. The Z Cam shell demonstrates that at least some dwarf novae must have undergone classical nova eruptions in the past, and that at least some classical novae become dwarf novae long after their nova thermonuclear outbursts, in accord with the hibernation scenario of cataclysmic binaries. Here we report the detection of a fragmented “shell,” 3 arcmin in diameter, surrounding the dwarf nova AT Cancri. This second discovery demonstrates that nova shells surrounding Z Cam-type dwarf novae cannot be very rare. -
A Review of the Disc Instability Model for Dwarf Novae, Soft X-Ray Transients and Related Objects a J.M
A review of the disc instability model for dwarf novae, soft X-ray transients and related objects a J.M. Hameury aObservatoire Astronomique de Strasbourg ARTICLEINFO ABSTRACT Keywords: I review the basics of the disc instability model (DIM) for dwarf novae and soft-X-ray transients Cataclysmic variable and its most recent developments, as well as the current limitations of the model, focusing on the Accretion discs dwarf nova case. Although the DIM uses the Shakura-Sunyaev prescription for angular momentum X-ray binaries transport, which we know now to be at best inaccurate, it is surprisingly efficient in reproducing the outbursts of dwarf novae and soft X-ray transients, provided that some ingredients, such as irradiation of the accretion disc and of the donor star, mass transfer variations, truncation of the inner disc, etc., are added to the basic model. As recently realized, taking into account the existence of winds and outflows and of the torque they exert on the accretion disc may significantly impact the model. I also discuss the origin of the superoutbursts that are probably due to a combination of variations of the mass transfer rate and of a tidal instability. I finally mention a number of unsolved problems and caveats, among which the most embarrassing one is the modelling of the low state. Despite significant progresses in the past few years both on our understanding of angular momentum transport, the DIM is still needed for understanding transient systems. 1. Introduction tems using Doppler tomography techniques (Steeghs et al. 1997; Marsh and Horne 1988; see also Marsh and Schwope Dwarf novae (DNe) are cataclysmic variables (CV) that 2016 for a recent review). -
The SAI Catalog of Supernovae and Radial Distributions of Supernovae
Astronomy Letters, Vol. 30, No. 11, 2004, pp. 729–736. Translated from Pis’ma v Astronomicheski˘ı Zhurnal, Vol. 30, No. 11, 2004, pp. 803–811. Original Russian Text Copyright c 2004 by Tsvetkov, Pavlyuk, Bartunov. TheSAICatalogofSupernovaeandRadialDistributions of Supernovae of Various Types in Galaxies D. Yu. Tsvetkov*, N.N.Pavlyuk**,andO.S.Bartunov*** Sternberg Astronomical Institute, Universitetski ˘ı pr. 13, Moscow, 119992 Russia Received May 18, 2004 Abstract—We describe the Sternberg Astronomical Institute (SAI)catalog of supernovae. We show that the radial distributions of type-Ia, type-Ibc, and type-II supernovae differ in the central parts of spiral galaxies and are similar in their outer regions, while the radial distribution of type-Ia supernovae in elliptical galaxies differs from that in spiral and lenticular galaxies. We give a list of the supernovae that are farthest from the galactic centers, estimate their relative explosion rate, and discuss their possible origins. c 2004MAIK “Nauka/Interperiodica”. Key words: astronomical catalogs, supernovae, observations, radial distributions of supernovae. INTRODUCTION be found on the Internet. The most complete data are contained in the list of SNe maintained by the Cen- In recent years, interest in studying supernovae (SNe)has increased signi ficantly. Among other rea- tral Bureau of Astronomical Telegrams (http://cfa- sons, this is because SNe Ia are used as “standard www.harvard.edu/cfa/ps/lists/Supernovae.html)and candles” for constructing distance scales and for cos- the electronic version of the Asiago catalog mological studies, and because SNe Ibc may be re- (http://web.pd.astro.it/supern). lated to gamma ray bursts. -
Present Status and Future Directions of the EVN
http://www.evlbi.org/ Present status and future directions of the EVN Michael Lindqvist, Onsala Space Observatory Zsolt Paragi, JIVE Onsala Space Observatory http://www.evlbi.org/ Thanks Tasso! Onsala Space Observatory http://www.evlbi.org/ VLBI science • Radio jet & black hole physics • Radio source evolution • Astrometry • Galactic and extra-galactic masers • Gravitational lenses • Supernovae and gamma-ray-burst studies • Nearby and distant starburst galaxies • Nature of faint radio source population • HI absorption studies in AGN • Space science VLBI • Transients • SETI Onsala Space Observatory http://www.evlbi.org/ Description of the EVN • The European VLBI Network (EVN) was formed in 1980. Today it includes 15 major institutes, including the Joint Institute for VLBI ERIC, JIVE • JIVE operates EVN correlator. JIVE is also involved in supporting EVN users and operations of EVN as a facility. JIVE has officially been established as an European Research Infrastructure Consortium (ERIC). • The EVN operates an “open sky” policy • No standing centralised budget for the EVN - distributed European facility Onsala Space Observatory http://www.evlbi.org/ The network Onsala Space Observatory http://www.evlbi.org/ EVN and e-VLBI From tape reel to intercontinental light paths • Pieces falling into place around 2003: – Introduction of Mark5 recording system (game changer) by Haystack Observatory – Emergence of high bandwidth optical fibre networks e-VLBI JIVE Hot scienceJIVE • The development of e-VLBI has been spearheaded by the JIVE/EVN (EXPReS, Garrett) • In this way, the EVN/JIVE is a recognized SKA pathfinder Onsala Space Observatory http://www.evlbi.org/ e-VLBI - rapid turn-around • e-VLBI has made rapid turn-around possible – X-ray, γ-ray binaries in flaring states (including novae) – AGN γ-ray outbursts — locus of VHE emission – Other high-energy flaring (e.g., Crab) – Outbursts in Mira variables (spectral-line) – Just-exploded GRBs, SNe – Binaries (incl. -
V2487 Oph 1998: a Post Nova in an Intermediate Polar
EPJ Web of Conferences 64, 07002 (2014) DOI: 10.1051/epjconf/20146407002 C Owned by the authors, published by EDP Sciences, 2014 V2487 Oph 1998: a post nova in an intermediate polar Margarita Hernanz1;a 1Institute of Space Sciences - ICE (CSIC-IEEC), Campus UAB, Fac. Ciències, C5 par 2a pl., 08193 Bellaterra (Barcelona), Spain Abstract. V2487 Oph (Nova Oph 1998) is a classical nova that exploded in 1998. XMM-Newton observations performed between 2 and 9 years after the explosion showed emission related to restablished accretion, and indicative of a magnetic white dwarf. The spectrum looks like that of a cataclysmic variable of the intermediate polar type. Anyway, we don’t have yet a definitive confirmation of the intermediate polar character, through determination of spin and orbital periods. Although it is not the first nova exploding in a magnetic white dwarf, it is always challenging to reach explosive conditions when a stan- dard accretion disk can’t be formed, because of the magnetic field. In addition, V2487 Oph has been the first nova where a detection of X-rays - in the host binary system - has been reported prior to its eruption, in 1990 with the ROSAT satellite. V2487 Oph has been also detected in hard X-rays with INTEGRAL/IBIS and RXTE/PCA. Last but not least, V2487 Oph has been identified as a recurrent nova in 2008, since a prior eruption in 1900 has been reported through analysis of Harvard photographic plates. Therefore, it is expected to host a massive white dwarf and be a candidate for a type Ia supernova explo- sion. -
An Observational Argument Against Accretion in Magnetars
Astronomy & Astrophysics manuscript no. start c ESO 2020 September 30, 2020 An observational argument against accretion in magnetars V.Doroshenko1; 2, A. Santangelo1, V. F. Suleimanov1; 2; 3, and S. S. Tsygankov4; 2 1 Institut für Astronomie und Astrophysik, Sand 1, 72076 Tübingen, Germany 2 Space Research Institute of the Russian Academy of Sciences, Profsoyuznaya Str. 84/32, Moscow 117997, Russia 3 Astronomy Department, Kazan (Volga region) Federal University, Kremlyovskaya str. 18, 420008 Kazan, Russia 4 Department of Physics and Astronomy, FI-20014 University of Turku, Finland September 30, 2020 ABSTRACT The phenomenology of anomalous X-ray pulsars is usually interpreted within the paradigm of very highly magnetized neutron stars, also known as magnetars. According to this paradigm, the persistent emission of anomalous X-ray pulsars (AXPs) is powered by the decay of the magnetic field. However, an alternative scenario in which the persistent emission is explained through accretion is also discussed in literature. In particular, AXP 4U 0142+61 has been suggested to be either an accreting neutron star or a white dwarf. Here, we rule out this scenario based on the the observed X-ray variability properties of the source. We directly compare the observed power spectra of 4U 0142+61 and of two other magnetars, 1RXS J170849.0−400910 and 1E 1841−045 with that of the X-ray pulsar 1A 0535+262, and of the intermediate polar GK Persei. In addition, we include a bright young radio pulsar PSR B1509-58 for comparison. We show that, unlike accreting sources, no aperiodic variability within the expected frequency range is observed in the power density spectrum of the magnetars and the radio pulsar. -
Introduction to Astronomical Photometry, Second Edition
This page intentionally left blank Introduction to Astronomical Photometry, Second Edition Completely updated, this Second Edition gives a broad review of astronomical photometry to provide an understanding of astrophysics from a data-based perspective. It explains the underlying principles of the instruments used, and the applications and inferences derived from measurements. Each chapter has been fully revised to account for the latest developments, including the use of CCDs. Highly illustrated, this book provides an overview and historical background of the subject before reviewing the main themes within astronomical photometry. The central chapters focus on the practical design of the instruments and methodology used. The book concludes by discussing specialized topics in stellar astronomy, concentrating on the information that can be derived from the analysis of the light curves of variable stars and close binary systems. This new edition includes numerous bibliographic notes and a glossary of terms. It is ideal for graduate students, academic researchers and advanced amateurs interested in practical and observational astronomy. Edwin Budding is a research fellow at the Carter Observatory, New Zealand, and a visiting professor at the Çanakkale University, Turkey. Osman Demircan is Director of the Ulupınar Observatory of Çanakkale University, Turkey. Cambridge Observing Handbooks for Research Astronomers Today’s professional astronomers must be able to adapt to use telescopes and interpret data at all wavelengths. This series is designed to provide them with a collection of concise, self-contained handbooks, which covers the basic principles peculiar to observing in a particular spectral region, or to using a special technique or type of instrument. The books can be used as an introduction to the subject and as a handy reference for use at the telescope, or in the office.