Qt56k8q6qx.Pdf
Total Page:16
File Type:pdf, Size:1020Kb
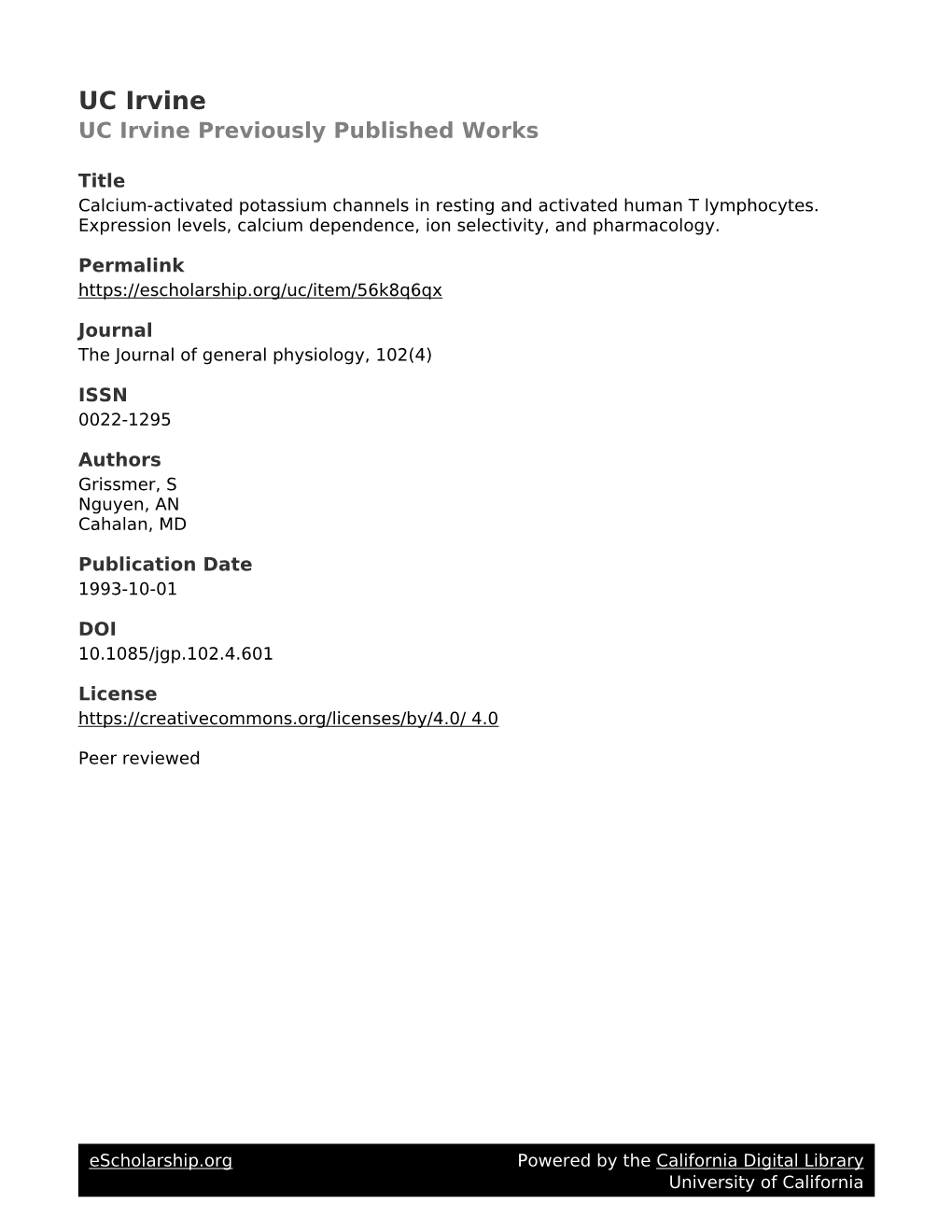
Load more
Recommended publications
-
Charybdotoxin and Noxiustoxin, Two Homologous Peptide Inhibitors of the K+(Ca2+) Channel
View metadata, citation and similar papers at core.ac.uk brought to you by CORE provided by Elsevier - Publisher Connector Volume 226, number 2, 280-284 FEB 05447 January 1988 Charybdotoxin and noxiustoxin, two homologous peptide inhibitors of the K+(Ca2+) channel Hector H. Valdivia*, Jeffrey S. Smith*, Brian M. Martin+, Roberto Coronado* and Lourival D. Possani*’ *Department of Physiology and Molecular Biophysics, Baylor College of Medicine, I Baylor Plaza, Houston, TX 77030, +National Institute of Mental Health, Molecular Neurogenetics Unit, Clinical Neuroscience Branch, Building IO 3016. NIH, Bethesda, MD 20892, USA and “Departamento de Bioquimica, Centro de Investigation sobre Ingenieria Genetica y Biotecnologia. Universidad National Autonoma de Mexico. Apartado Postal 510-3 Cuernavaca, Morelos 62271, Mexico Received 30 October 1987 We show that noxiustoxin (NTX), like charybdotoxin (CTX) described by others, affects CaZt-activated K+ channels of skeletal muscle (K+(Ca2+) channels). Chemical characterization of CTX shows that it is similar to NTX. Although the amino-terminal amino acid of CTX is not readily available, the molecule was partially sequenced after CNBr cleavage. A decapeptide corresponding to the C-terminal region of NTX shows 60% homology to that of CTX, maintaining the cysteine residues at the same positions. While CTX blocks the K+(Ca2+) channels with a & of 1-3 nM, for NTX it is approx. 450 nM. Both peptides can interact simultaneously with the same channel. NTX and CTX promise to be good tools for channel isolation. -
K Channels As Targets for Specific Immunomodulation
Review TRENDS in Pharmacological Sciences Vol.25 No.5 May 2004 K1 channels as targets for specific immunomodulation K. George Chandy1, Heike Wulff2, Christine Beeton1, Michael Pennington3, George A. Gutman1 and Michael D. Cahalan1 1Department of Physiology and Biophysics, University of California, Irvine, CA 92697, USA 2Department of Pharmacology and Toxicology, University of California, Davis, CA 95616, USA 3Bachem Bioscience, King of Prussia, PA 19406, USA 21 The voltage-gated Kv1.3 channel and the Ca -activated gene encodes the lymphocyte KV channel [8,9].An IKCa1 K1 channel are expressed in T cells in a distinct intermediate-conductance Ca2þ-activated Kþ channel pattern that depends on the state of lymphocyte acti- was identified in T cells in 1992 [10–12], and shown to vation and differentiation. The channel phenotype be a product of the KCNN4 (IKCa1, KCa3.1; http://www. changes during the progression from the resting to the iuphar-db.org/iuphar-ic/KCa.html) gene in 1997 [13]. activated cell state and from naı¨ve to effector memory Subsequent studies by our group identified calmodulin cells, affording promise for specific immunomodulatory as the Ca2þ sensor of the IKCa1 channel [14]. The salient actions of K1 channel blockers. In this article, we review features of both channels were summarized in a recent the functional roles of these channels in both naı¨ve cells review [15]. and memory cells, describe the development of selec- Following the discovery that Kþ channels are essential tive inhibitors of Kv1.3 and IKCa1 channels, and provide for T-cell function, several other Kþ channels have been a rationale for the potential therapeutic use of these implicated in the proliferation of a wide variety of normal inhibitors in immunological disorders. -
Fast K+ Currents from Cerebellum Granular Cells Are Completely Blocked by a Peptide Puri¢Ed from Androctonus Australis Garzoni
Biochimica et Biophysica Acta 1468 (2000) 203^212 www.elsevier.com/locate/bba Fast K currents from cerebellum granular cells are completely blocked by a peptide puri¢ed from Androctonus australis Garzoni scorpion venom Marzia Pisciotta a, Fredy I. Coronas b, Carlos Bloch c, Gianfranco Prestipino a;1;*, Lourival D. Possani b;1;2 a Istituto di Cibernetica e Bio¢sica, C.N.R., via De Marini 6, 16149 Genova, Italy b Biotechnology Institute-UNAM, Av. Universidad 2001, Cuernavaca 62210, Mexico c EMBRAPA/Cenargen, P.O. Box 02372, Brasilia, DF, Brazil Received 22 February 2000; received in revised form 25 May 2000; accepted 7 June 2000 Abstract A novel peptide was purified from the venom of the scorpion Androctonus australis Garzoni (abbreviated Aa1, corresponding to the systematic number alpha KTX4.4). It contains 37 amino acid residues, has a molecular mass of 3850 Da, is closely packed by three disulfide bridges and a blocked N-terminal amino acid. This peptide selectively affects the K currents recorded from cerebellum granular cells. Only the fast activating and inactivating current, with a kinetics similar to IA-type current, is completely blocked by the addition of low micromolar concentrations (Ki value of 150 nM) of peptide Aa1 to the external side of the cell preparation. The blockade is partially reversible in our experimental conditions. Aa1 blocks the channels in both the open and the closed states. The blockage is test potential independent and is not affected by changes in the holding potential. The kinetics of the current are not affected by the addition of Aa1 to the preparation; it means that the block is a simple `plugging mechanism', in which a single toxin molecule finds a specific receptor site in the external vestibule of the K channel and thereby occludes the outer entry to the K conducting pore. -
The Electronic Structure and Dipole Moment of Charybdotoxin, a Scorpion Venom Peptide with K+ Channel Blocking Activity
The electronic structure and dipole moment of charybdotoxin, a scorpion venom peptide with K+ channel blocking activity Fabio Pichierri* G-COE Laboratory, Department of Applied Chemistry, Graduate School of Engineering, Tohoku University, Aoba-yama 6-6-07, Sendai 980-8579, Japan [v1, 21 May 2010] Abstract The electronic structure of charybdotoxin (ChTX), a scorpion venom peptide that is known to act as a potassium channel blocker, is investigated with the aid of quantum mechanical calculations. The dipole moment vector (=145 D) of ChTX can be stirred by the full length KcsA potassium channel’s macrodipole (=403 D) thereby assuming the proper orientation before binding the ion channel on the cell surface. The localization of the frontier orbitals of ChTX has been revealed for the first time. HOMO is localized on Trp14 while the three lowest-energy MOs (LUMO, LUMO+1, and LUMO+2) are localized on the three disulfide bonds that characterize this pepetide. An effective way to engineer the HOMO-LUMO (H-L) gap of ChTX is that of replacing its Trp14 residue with Ala14 whereas deletion of the LUMO-associated disulfide bond with the insertion of a pair of L--aminobutyric acid residues does not affect the H-L energy gap. Keywords: Charybdotoxin; Scorpion venom peptide; Potassium channel; Dipole moment; Electronic structure; Quantum chemistry * Corresponding author. Tel. & Fax: +81-22-795-4132 E-mail address: [email protected] (F. Pichierri) 1 1. Introduction The venom of scorpions contains a pool of several globular peptides (mini-proteins) which have the ability to bind a variety of ion (Na+, K+, Ca2+) channels located on the cell surface of the organism under attack [1-3]. -
Slow Inactivation in Voltage Gated Potassium Channels Is Insensitive to the Binding of Pore Occluding Peptide Toxins
Biophysical Journal Volume 89 August 2005 1009–1019 1009 Slow Inactivation in Voltage Gated Potassium Channels Is Insensitive to the Binding of Pore Occluding Peptide Toxins Carolina Oliva, Vivian Gonza´lez, and David Naranjo Centro de Neurociencias de Valparaı´so, Facultad de Ciencias, Universidad de Valparaı´so, Valparaı´so, Chile ABSTRACT Voltage gated potassium channels open and inactivate in response to changes of the voltage across the membrane. After removal of the fast N-type inactivation, voltage gated Shaker K-channels (Shaker-IR) are still able to inactivate through a poorly understood closure of the ion conduction pore. This, usually slower, inactivation shares with binding of pore occluding peptide toxin two important features: i), both are sensitive to the occupancy of the pore by permeant ions or tetraethylammonium, and ii), both are critically affected by point mutations in the external vestibule. Thus, mutual interference between these two processes is expected. To explore the extent of the conformational change involved in Shaker slow inactivation, we estimated the energetic impact of such interference. We used kÿconotoxin-PVIIA (kÿPVIIA) and charybdotoxin (CTX) peptides that occlude the pore of Shaker K-channels with a simple 1:1 stoichiometry and with kinetics 100-fold faster than that of slow inactivation. Because inactivation appears functionally different between outside-out patches and whole oocytes, we also compared the toxin effect on inactivation with these two techniques. Surprisingly, the rate of macroscopic inactivation and the rate of recovery, regardless of the technique used, were toxin insensitive. We also found that the fraction of inactivated channels at equilibrium remained unchanged at saturating kÿPVIIA. -
Ion Channels
UC Davis UC Davis Previously Published Works Title THE CONCISE GUIDE TO PHARMACOLOGY 2019/20: Ion channels. Permalink https://escholarship.org/uc/item/1442g5hg Journal British journal of pharmacology, 176 Suppl 1(S1) ISSN 0007-1188 Authors Alexander, Stephen PH Mathie, Alistair Peters, John A et al. Publication Date 2019-12-01 DOI 10.1111/bph.14749 License https://creativecommons.org/licenses/by/4.0/ 4.0 Peer reviewed eScholarship.org Powered by the California Digital Library University of California S.P.H. Alexander et al. The Concise Guide to PHARMACOLOGY 2019/20: Ion channels. British Journal of Pharmacology (2019) 176, S142–S228 THE CONCISE GUIDE TO PHARMACOLOGY 2019/20: Ion channels Stephen PH Alexander1 , Alistair Mathie2 ,JohnAPeters3 , Emma L Veale2 , Jörg Striessnig4 , Eamonn Kelly5, Jane F Armstrong6 , Elena Faccenda6 ,SimonDHarding6 ,AdamJPawson6 , Joanna L Sharman6 , Christopher Southan6 , Jamie A Davies6 and CGTP Collaborators 1School of Life Sciences, University of Nottingham Medical School, Nottingham, NG7 2UH, UK 2Medway School of Pharmacy, The Universities of Greenwich and Kent at Medway, Anson Building, Central Avenue, Chatham Maritime, Chatham, Kent, ME4 4TB, UK 3Neuroscience Division, Medical Education Institute, Ninewells Hospital and Medical School, University of Dundee, Dundee, DD1 9SY, UK 4Pharmacology and Toxicology, Institute of Pharmacy, University of Innsbruck, A-6020 Innsbruck, Austria 5School of Physiology, Pharmacology and Neuroscience, University of Bristol, Bristol, BS8 1TD, UK 6Centre for Discovery Brain Science, University of Edinburgh, Edinburgh, EH8 9XD, UK Abstract The Concise Guide to PHARMACOLOGY 2019/20 is the fourth in this series of biennial publications. The Concise Guide provides concise overviews of the key properties of nearly 1800 human drug targets with an emphasis on selective pharmacology (where available), plus links to the open access knowledgebase source of drug targets and their ligands (www.guidetopharmacology.org), which provides more detailed views of target and ligand properties. -
Proteins, Peptides, and Amino Acids
Proteins, Peptides, and Amino Acids Chandra Mohan, Ph.D. Calbiochem-Novabiochem Corp., San Diego, CA The Chemical Nature of Amino Acids Peptides and polypeptides are polymers of α-amino acids. There are 20 α-amino acids that make-up all proteins of biological interest. The α-amino acids in peptides and proteins α consist of a carboxylic acid (-COOH) and an amino (-NH2) functional group attached to the same tetrahedral carbon atom. This carbon is known as the -carbon. The type of R- group attached to this carbon distinguishes one amino acid from another. Several other amino acids, also found in the body, may not be associated with peptides or proteins. These non-protein-associated amino acids perform specialized functions. Some of the α-amino acids found in proteins are also involved in other functions in the body. For example, tyrosine is involved in the formation of thyroid hormones, and glutamate and aspartate act as neurotransmitters at fast junctions. R Amino acids exist in either D- or L- enantiomorphs or stereoisomers. The D- and L-refer to the absolute confirmation of optically active compounds. With the exception of glycine, all other amino acids are mirror images that can not be superimposed. Most of the amino acids found in nature are of the L-type. Hence, eukaryotic proteins are always composed of L-amino acids although D-amino acids are found in bacterial cell walls and in some peptide antibiotics. All biological reactions occur in an aqueous phase. Hence, it is important to know how the R-group of any given amino acid dictates the structure-function relationships of peptides and proteins in solution. -
United States Patent (19) 11 Patent Number: 4,929,718 Possani Et Al
United States Patent (19) 11 Patent Number: 4,929,718 Possani et al. 45 Date of Patent: May 29, 1990 54 SYNTHETIC NOXIUSTOXIN RELATED Fletcher B. Taylor, Jr., et al., Thrombosis Research, PEPTDES “Clots Lysis: Effects of Protein C''', 37: 639-649, 1985. Hector H. Valdiva, et al., Elsevier Science Publishers 75 Inventors: Lourival D. P. Possani; Georgina B. B.V. (Biomedical Divison) “FEBS Letters', 226(2); Gurrola, both of Cuernavaca; Marco 280-284, 1988. A. A. C. Bayon; Maria B. Sitges, both E. Carbone, et al., European Journal of Physiology, of Mexico, all of Mexico "Blocking of the Squid Axon L-- Channel by Noxius 73 Assignee: Universidad Nacional Autonoma De toxin', 408; 423-431, 1987. Mexico, Dependence, Mexico Maria Sitges, et al., The Journal of Neuroscience, 21 Appl. No.: 132,169 “NTX-Induced Transmitter Release and K-- Permea bility’, 6(6): 1570-1574, 1986. 22 Filed: Dec. 14, 1987 51) Int. Cl. ........................... C07K 7/06; C07K7/08 Primary Examiner-Lester L. Lee 52 U.S. Cl. .................................... 530/326; 530/328; Attorney, Agent, or Firm-Fleit, Jacobson, Cohn, Price, 530/324; 530/858 Holman & Stern 58 Field of Search ............... 530/324, 858, 328, 326; 57 ABSTRACT 514/12 The invention relates to a novel immunogenic synthetic 56) References Cited peptide that is capable of specifically blocking potas U.S. PATENT DOCUMENTS sium channels of excitable membranes and inducing immunity against an effective lethal dose of Noxius 4,499,080 2/1985 Duflot et al. ........................ 530/324 toxin. The invention also relates to the discovery that OTHER PUBLICATIONS the immunogenic and blocking properties of the peptide L. -
(K+) Channels: a Historical Overview of Peptide Bioengineering
Toxins 2012, 4, 1082-1119; doi:10.3390/toxins4111082 OPEN ACCESS toxins ISSN 2072-6651 www.mdpi.com/journal/toxins Review Scorpion Toxins Specific for Potassium (K+) Channels: A Historical Overview of Peptide Bioengineering Zachary L. Bergeron and Jon-Paul Bingham * Department of Molecular Biosciences and Bioengineering, College of Tropical Agriculture and Human Resources, University of Hawaii at Manoa, Honolulu, HI 96822, USA; E-Mail: [email protected] * Author to whom correspondence should be addressed; E-Mail: [email protected]; Tel.: +1-808-956-4864; Fax: +1-808-956-3542. Received: 14 September 2012; in revised form: 22 October 2012 / Accepted: 23 October 2012 / Published: 1 November 2012 Abstract: Scorpion toxins have been central to the investigation and understanding of the physiological role of potassium (K+) channels and their expansive function in membrane biophysics. As highly specific probes, toxins have revealed a great deal about channel structure and the correlation between mutations, altered regulation and a number of human pathologies. Radio- and fluorescently-labeled toxin isoforms have contributed to localization studies of channel subtypes in expressing cells, and have been further used in competitive displacement assays for the identification of additional novel ligands for use in research and medicine. Chimeric toxins have been designed from multiple peptide scaffolds to probe channel isoform specificity, while advanced epitope chimerization has aided in the development of novel molecular therapeutics. Peptide backbone cyclization has been utilized to enhance therapeutic efficiency by augmenting serum stability and toxin half-life in vivo as a number of K+-channel isoforms have been identified with essential roles in disease states ranging from HIV, T-cell mediated autoimmune disease and hypertension to various cardiac arrhythmias and Malaria. -
Mullmann Et Al 200
Biochemistry 2001, 40, 10987-10997 10987 Insights into R-Κ Toxin Specificity for K+ Channels Revealed through Mutations in Noxiustoxin† Theodore J. Mullmann,‡ Katherine T. Spence,§ Nathan E. Schroeder,‡ Valerie Fremont,‡ Edward P. Christian,§ and Kathleen M. Giangiacomo*,‡ Department of Biochemistry, Temple UniVersity School of Medicine, 3420 North Broad Street, Philadelphia, PennsylVania 19140, and Department of Neuroscience, Astra-Zeneca Pharmaceuticals, 1800 Concord Pike, Wilmington, Delaware 19850 ReceiVed February 2, 2001; ReVised Manuscript ReceiVed July 3, 2001 ABSTRACT: Noxiustoxin (NxTX) displays an extraordinary ability to discriminate between large conductance, calcium-activated potassium (maxi-K) channels and voltage-gated potassium (Kv1.3) channels. To identify features that contribute to this specificity, we constructed several NxTX mutants and examined their effects on whole cell current through Kv1.3 channels and on current through single maxi-K channels. Recombinant NxTX and the site-specific mutants (P10S, S14W, A25R, A25∆) all inhibited Kv1.3 channels with Kd values of 6, 30, 0.6, 112, and 166 nM, respectively. In contrast, these same NxTX mutants had no effect on maxi-K channel activity with estimated Kd values exceeding 1 mM. To examine the role of the R-carbon backbone in binding specificity, we constructed four NxTX chimeras, which altered the backbone length and the R/â turn. For each of these chimeras, six amino acids comprising the R/â turn in iberiotoxin (IbTX) replaced the corresponding seven amino acids in NxTX (NxTX-YGSSAGA21-27-FGVDRG21-26). The chimeras differed in length of N- and C-terminal residues and in critical contact residues. In contrast to NxTX and its site-directed mutants, all of these chimeras inhibited single maxi-K channels. -
Purification, Sequence, and Model Structure of Charybdotoxin, a Potent
Proc. Nati. Acad. Sci. USA Vol. 85, pp. 3329-3333, May 1988 Biochemistry Purification, sequence, and model structure of charybdotoxin, a potent selective inhibitor of calcium-activated potassium channels (ion-channel blocker/scorpion toxin/sequence homologies/snake neurotoxin) GUILLERMO GIMENEZ-GALLEGO*t, MANUEL A. NAVIAt, JOHN P. REUBEN§, GEORGE M. KATZ§, GREGORY J. KACZOROWSKI§, AND MARIA L. GARCIA§¶ Departments of *Growth Factor Research, tBiophysics, and Membrane Biochemistry, Merck Sharp & Dohme Research Laboratories, P.O. Box 2000, Rahway, NJ 07065 Communicated by Edward M. Scolnick, January 6, 1988 ABSTRACT Charybdotoxin (ChTX), a protein present in crepancies were noted in the determination of the molecular the venom of the scorpion Leiurus quinquestriatus var. he- mass of ChTX based on amino acid composition and electro- braeus, has been purified to homogeneity by a combination of phoretic mobility of the protein, which could be explained by ion-exchange and reversed-phase chromatography. Polyacryl- inhomogeneity of the preparation. amide gel electrophoresis, amino acid analysis, and complete The present study describes the purification of ChTX to amino acid sequence determination of the pure protein reveal homogeneity, the biological activity ofthe pure toxin, and the that it consists ofa single polypeptide chain of4.3 kDa. Purified chemical characterization of this peptide in terms of amino ChTX is a potent and selective inhibitor of the -220-pS acid composition and sequence. The primary structure Ca21 -activated K+ channel present in GH3 anterior pituitary uniquely identifies this molecule and reveals its similarity to cells and primary bovine aortic smooth muscle cells. The toxin other toxins of species as phylogenetically distant as snakes reversibly blocks channel activity by interacting at the external and marine worms. -
European Academic Research
EUROPEAN ACADEMIC RESEARCH Vol. IV, Issue 1/ April 2016 Impact Factor: 3.4546 (UIF) ISSN 2286-4822 DRJI Value: 5.9 (B+) www.euacademic.org Margatoxin (MgTX) and Its Effect on Immune Response and Disease Development SALAUDDIN AL AZAD MS student Biotechnology and Genetic Engineering Discipline Khulna University, Bangladesh SAYEED SHAHRIYAR1 MS student Department of Biotechnology Bangladesh Agricultural University Mymenshingh, Bangladesh KANAK JYOTI MONDAL Registrar, Medicine Khulna Medical College Hospital, Khulna, Bangladesh Abstract: Margatoxin (MgTX) is a protein molecule which is generated from Central American Bark Scorpions (also called Centruroides margatitatus) as their defense agent, is very selective to the inhibition of Kv1.3 voltage-dependent potassium channel through the miss regulation of GLUT4 trafficking on the cell membrane via Ca2+ dependent mechanism. Consequently insulin production mechanism is hampered directly, a panic to the diabetic patients. The toxin molecule is the concern of many immunologists all over United States of America because there is seldom drugs are available to combat against the toxin. In the following passages the signs and symptoms of margatoxin invasion, biosynthesis, mechanism of the immune suppression, clinical aspects of MgTX and the future of drug design against the protein molecule are described stepwise. Synthetic MgTX 1 Corresponding author: [email protected] 40 Salauddin Al Azad, Sayeed Shahriyar, Kanak Jyoti Mondal- Margatoxin (MgTX) and Its Effect on Immune Response and Disease Development gene development and plasmid designing to insert it into E. coli for manipulating the desired amount of MgTX peptide is a master blessing of biotechnology where the main options of molecular or nanomedicine development lies on the structural modification analysis of the 39 amino acid sequence of the toxic protein molecule.