Hydrodynamical Models of Cometary HII Regions
Total Page:16
File Type:pdf, Size:1020Kb
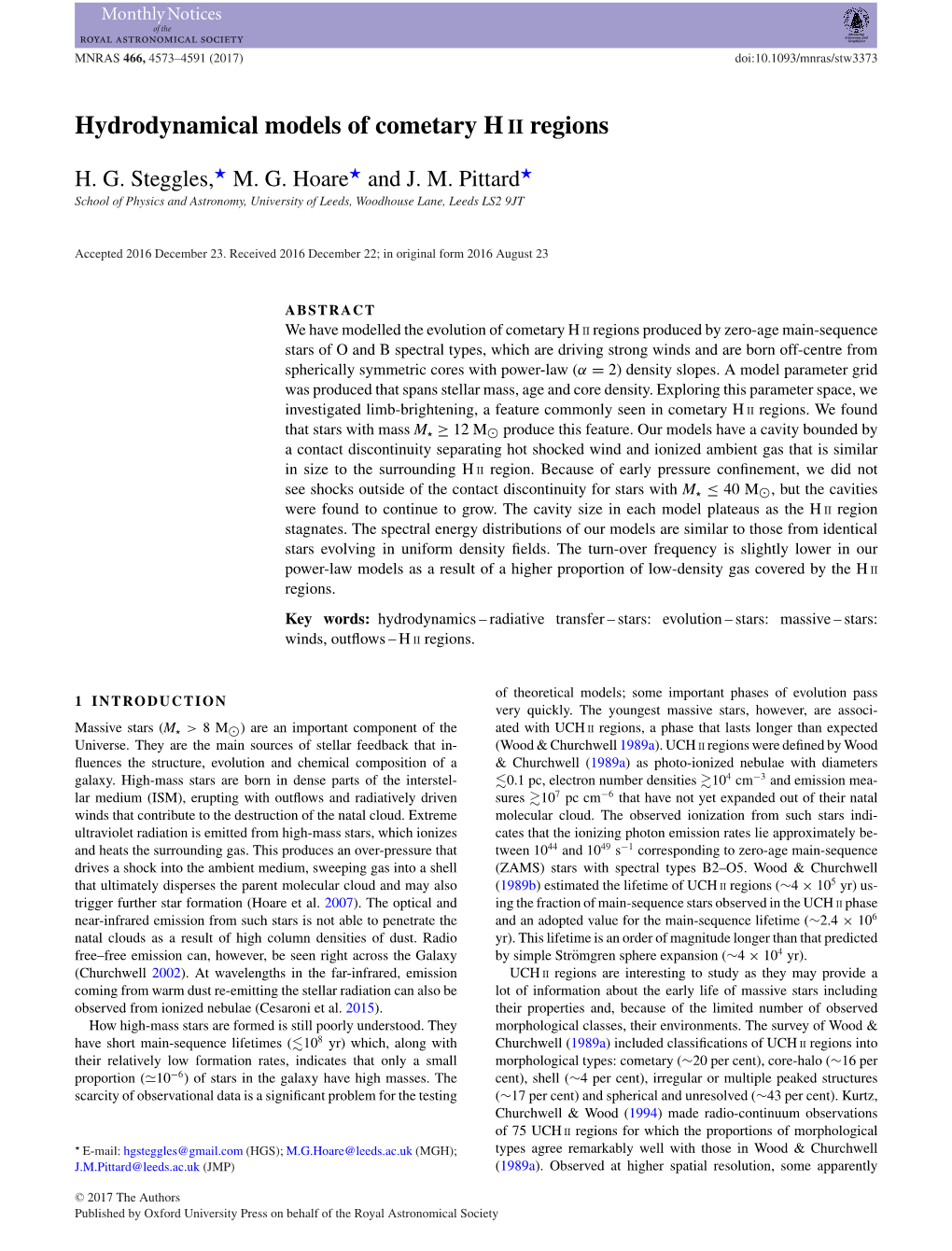
Load more
Recommended publications
-
Mid-Infrared Polarimetry: Insights Into Magnetic Fields and Dust Grain Properties in Young Stellar Objects and Protoplanetary Disks
MID-INFRARED POLARIMETRY: INSIGHTS INTO MAGNETIC FIELDS AND DUST GRAIN PROPERTIES IN YOUNG STELLAR OBJECTS AND PROTOPLANETARY DISKS By HAN ZHANG A DISSERTATION PRESENTED TO THE GRADUATE SCHOOL OF THE UNIVERSITY OF FLORIDA IN PARTIAL FULFILLMENT OF THE REQUIREMENTS FOR THE DEGREE OF DOCTOR OF PHILOSOPHY UNIVERSITY OF FLORIDA 2018 ⃝c 2018 Han Zhang When I look at your heavens, the work of your fingers, the moon and the stars, which you have set in place, what is mankind that you are mindful of them, human beings that you care for them? { Psalm 8:3-4 ACKNOWLEDGMENTS Firstly, I would like to thank my advisor, Charles M. Telesco, for his patience, guidance, and generous financial supports for the past five years. It is a great privilege for me to work with Charlie and be part of the CanariCam science team. I want to thank all my collaborators, without your contributions, I can not finish the work presented in this thesis. I am particularly thankful to Dan Li, Eric Pantin, Aigen Li, Christopher M. Wright, Peter Barnes, and Naib´ıMari~nas.Thank you for your insightful discussions and comments. I thank my fellow graduate students here at University of Florida. My great classmates Rebecca, Nolan, Chen and Pekki, I remember the days we worked on the homework and projects together. I want to thank Jingzhe, my first-year roommate at UF, and Xiao, my driving coach. I want to thank Emily, Wenli, Chutipong, Shuo, Nahathai, Hanna, Tahlia, Yinan, Amanda, Rachel, Alan, Krittapas, Ben Wu, and Billy. Thank you all for your company and love. -
THE STAR FORMATION NEWSLETTER an Electronic Publication Dedicated to Early Stellar Evolution and Molecular Clouds
THE STAR FORMATION NEWSLETTER An electronic publication dedicated to early stellar evolution and molecular clouds No. 76 — 26 January 1999 Editor: Bo Reipurth ([email protected]) Abstracts of recently accepted papers Deuterium fractionation and the degree of ionization in the R Coronae Australis molec- ular cloud core I.M. Anderson1,2, P. Caselli3, L.K. Haikala1,4, J. Harju1 1 Observatory, P.O. Box 14, FIN-00014 University of Helsinki, Finland 2 Watson Wyatt Partners, 86 Station Road,Redhill, Surrey RH1 1PL, England 3 Osservatorio Astrofisico di Arcetri, Largo E. Fermi 5, I-50125 Firenze, Italy 4 Swedish-ESO Submillimetre Telescope, European Southern Observatory, Casilla 19001, Santiago 19, Chile E-mail contact: [email protected].fi The fractionation of D and 13C in HCO+ was investigated in the R Coronae Australis molecular cloud core. The distributions of H13CO+ and DCO+ were found to be morphologically similar but their column density maxima were found to lie in different locations. The H13CO+/HC18O+ abundance ratio was found to vary little from 10 within the mapped region, in excellent agreement with the 13CO/C18O abundance ratios derived earlier towards the cloud by Harjunp¨a¨a& Mattila (1996). This corroborates the close relationship between HCO+ and CO predicted by the chemistry models. The DCO+/HCO+ abundance ratio ranges from 0.006 to 0.04, being lowest towards two locations near the embedded infrared source IRS 7 where the kinetic temperature, as derived from methyl acetylene (CH3CCH) observations, is somewhat elevated. The variation of the degree of deuterium fractionation within the core is due to an increase in the kinetic temperature near the cluster of newly born stars. -
Gas Kinematics in the HII Regions G351. 69-1.15 and G351. 63-1.25
Mon. Not. R. Astron. Soc. 000, 1–?? () Printed 18 October 2018 (MN LATEX style file v2.2) Gas Kinematics in the H II regions G351.69–1.15 and G351.63–1.25 V. S. Veena1⋆, S. Vig1, A. Tej1, N. G. Kantharia2, S. K. Ghosh2 1Indian Institute of Space Science and Technology, Thiruvananthapuram, 695 547, India 2National Centre for Radio Astrophysics (NCRA-TIFR), Pune, 411 007, India ABSTRACT We probe the structure and kinematics of two neighbouringH II regions identified as cometary and bipolar,using radio recombinationlines (RRL). The H172α RRLs fromthese H II regions: G351.69–1.15 and G351.63–1.25, are mapped using GMRT, India. We also detect carbon RRLs C172α towards both these regions. The hydrogen RRLs display the effects of pressure and dynamical broadening in the line profiles, with the dynamical broadening (∼ 15 km/s) playing a major role in the observed profile of G351.69–1.15. We investigate the kinematics of molecular gas species towards this H II region from the MALT90 pilot survey. The molec- ular gas is mostly distributed towards the north and north-west of the cometary head. The molecular line profiles indicate signatures of turbulence and outflow in this region. The ion- ized gas at the cometary tail is blue shifted by ∼8 km/s with respect to the ambient molecular cloud, consistent with the earlier proposed champagne flow scenario. The relative velocity of ∼5 km/s between the northern and southern lobes of the bipolar H II region G351.63–1.25 is consistent with the premise that the bipolar morphology is a result of the expanding ionized lobes within a flat molecular cloud. -
The Dynamics of Ultracompact HII Regions
MNRAS 438, 1335–1354 (2014) doi:10.1093/mnras/stt2278 Advance Access publication 2013 December 19 The dynamics of ultracompact H II regions Nathaniel Roth,1‹ Steven W. Stahler2 and Eric Keto3 1Department of Physics, University of California, Berkeley, CA 94720, USA 2Department of Astronomy, University of California, Berkeley, CA 94720, USA 3Harvard–Smithsonian Center for Astrophysics, 60 Garden Street, Cambridge, MA 02138, USA Accepted 2013 November 22. Received 2013 November 18; in original form 2013 October 4 ABSTRACT Downloaded from Many ultracompact H II regions exhibit a cometary morphology in radio continuum emission. In such regions, a young massive star is probably ablating, through its ultraviolet radiation, the molecular cloud clump that spawned it. On one side of the star, the radiation drives an ionization front that stalls in dense molecular gas. On the other side, ionized gas streams outwards into the more rarefied environment. This wind is underpressured with respect to the http://mnras.oxfordjournals.org/ neutral gas. The difference in pressure draws in more cloud material, feeding the wind until the densest molecular gas is dissipated. Recent, time-dependent simulations of massive stars turning on within molecular gas show the system evolving in a direction similar to that just described. Here, we explore a semi-analytic model in which the wind is axisymmetric and has already achieved a steady state. Adoption of this simplified picture allows us to study the dependence of both the wind and its bounding ionization front on the stellar luminosity, the peak molecular density and the displacement of the star from the centre of the clump. -
Massive Stars: Their Environment and Formation
View metadata, citation and similar papers at core.ac.uk brought to you by CORE provided by CERN Document Server Massive stars: their environment and formation Guido Garay Departamento de Astronom´ıa, Universidad de Chile, Casilla 36-D, Santiago, Chile and Susana Lizano Instituto de Astronom´ıa, UNAM, Apdo. Postal 70-264, 04510 M´exico, D.F., M´exico Received ; accepted –2– ABSTRACT Cloud environment is thought to play a critical role in determining the mechanism of formation of massive stars. In this contribution we review the physical characteristics of the environment around recently formed massive stars. Particular emphasis is given to recent high angular resolution observations which have improved our knowledge of the physical conditions and kinematics of compact regions of ionized gas and of dense and hot molecular cores associated with luminous O and B stars. We will show that this large body of data, gathered during the last decade, has allowed significant progress in the understanding of the physical processes that take place during the formation and early evolution of massive stars. Subject headings: stars: formation — HII regions — ISM: clouds — ISM: kinematics and dynamics –3– 1. INTRODUCTION Massive stars in our Galaxy are born predominantly within the dense cores of giant molecular clouds. This premise is strongly supported by a wealth of observations which show that hallmarks of newly formed massive stars, such as compact regions of ionized gas, are intimately associated with warm and dense regions of molecular gas (Churchwell, Walmsley, & Cesaroni 1990; Cesaroni et al. 1991; Plume, Jaffe, & Evans 1992). It remains unclear, however, how the actual formation of massive stars takes place. -
254 — 13 February 2014 Editor: Bo Reipurth ([email protected]) List of Contents
THE STAR FORMATION NEWSLETTER An electronic publication dedicated to early stellar/planetary evolution and molecular clouds No. 254 — 13 February 2014 Editor: Bo Reipurth ([email protected]) List of Contents The Star Formation Newsletter Interview ...................................... 3 My Favorite Object ............................ 5 Editor: Bo Reipurth [email protected] Perspective ................................... 10 Technical Editor: Eli Bressert Abstracts of Newly Accepted Papers .......... 13 [email protected] Abstracts of Newly Accepted Major Reviews . 54 Technical Assistant: Hsi-Wei Yen Dissertation Abstracts ........................ 60 [email protected] New Jobs ..................................... 61 Editorial Board Meetings ..................................... 63 Summary of Upcoming Meetings ............. 66 Joao Alves Alan Boss Short Announcements ........................ 67 Jerome Bouvier New Books ................................... 68 Lee Hartmann Thomas Henning Paul Ho Jes Jorgensen Charles J. Lada Cover Picture Thijs Kouwenhoven Michael R. Meyer This image shows the blueshifted outflow cav- Ralph Pudritz ity from the embedded quadruple Class I source Luis Felipe Rodr´ıguez L1551 IRS5 based on Hα and [SII] images obtained Ewine van Dishoeck with the Subaru telescope images. Two short jets, Hans Zinnecker HH 154, are seen to emerge from the source region to the upper left. The outflow cavity has burst The Star Formation Newsletter is a vehicle for through the front of the cloud, exposing the rich fast distribution of information of interest for as- and complex Herbig-Haro shock structures within. tronomers working on star and planet formation A few faint knots from the HH 30 jet (outside the and molecular clouds. You can submit material field) are visible at the top of the image. The for the following sections: Abstracts of recently field is about 7×8 arcmin, corresponding to about accepted papers (only for papers sent to refereed 0.30×0.35 pc at the assumed distance of 150 pc. -
Molecular Counterparts of Ultracompact HII Regions With
Molecular Counterparts of Ultracompact HII Regions with Extended Envelopes Kee-Tae Kim1,2 and Bon-Chul Koo2 ABSTRACT We have carried out 13CO J=1−0, CS, and C34S J=2−1 and J=3−2 line obser- vations of molecular clouds associated with 16 ultracompact (UC) HII regions with extended envelopes. The molecular clouds are the ones that give birth to rich stellar clusters and/or very massive (O7−O4) stars. Our data show that the clouds are very clumpy and of irregular morphology. They usually have much larger masses, velocity dispersions, and fractions of dense gas than molecular clouds that form early B or late O stars. This is compatible with earlier findings that more massive stars form in more massive cores. The IR luminosity-to-mass ratio has a mean value of 9 L⊙/M⊙ and is little correlated with the cloud mass. Most molecular clouds have star formation efficiencies (SFE’s) of 1%−2%. We find size-linewidth and size-density relations in the 0.4 −1.2 13 forms of ∆v ∝ D and n(H2) ∝ D . CO cores are in general associated with compact HII regions regardless of the presence of UC HII regions therein. In contrast, CS cores are preferentially associated with compact HII regions that contain UC HII regions. As with the fact that the compact HII regions containing UC HII regions are more compact than those not associated with UC HII regions, these indicate that the former may be in an earlier evolutionary phase than the latter. The diffuse ex- tended envelopes of HII regions often develop in the direction of decreasing molecular gas density. -
Download This Article in PDF Format
A&A 537, A149 (2012) Astronomy DOI: 10.1051/0004-6361/201117958 & c ESO 2012 Astrophysics NGC 3503 and its molecular environment N. U. Duronea1, J. Vasquez1,2,C.E.Cappa1,2, M. Corti1,2, and E. M. Arnal1,2 1 Instituto Argentino de Radioastronomia, CONICET, CCT-La Plata, CC 5, 1894 Villa Elisa, Argentina e-mail: [email protected] 2 Facultad de Ciencias Astronómicas y Geofísicas, Universidad Nacional de La Plata, Paseo del Bosque s/n, 1900 La Plata, Argentina Received 26 August 2011 / Accepted 14 November 2011 ABSTRACT Aims. We present a study of the molecular gas and interstellar dust distribution in the environs of the Hii region NGC 3503 associated with the open cluster Pis 17 with the aim of investigating the spatial distribution of the molecular gas linked to the nebula and achieving a better understanding of the interaction of the nebula and Pis 17 with their molecular environment. Methods. We based our study on 12CO(1−0) observations of a region of ∼0◦.6 in size obtained with the 4-m NANTEN telescope, unpublished radio continuum data at 4800 and 8640 MHz obtained with the ATCA telescope, radio continuum data at 843 MHz obtained from SUMSS, and available IRAS, MSX, IRAC-GLIMPSE, and MIPSGAL images. Results. We found a molecular cloud (Component 1) having a mean velocity of –24.7 km s−1, compatible with the velocity of the ionized gas, which is associated with the nebula and its surroundings. Adopting a distance of 2.9 ± 0.4 kpc, the total molecular mass 3 −3 yields (7.6 ± 2.1) × 10 M and density yields 400 ± 240 cm . -
Hydrodynamics of Cometary Compact HII Regions
Draft version November 9, 2018 Preprint typeset using LATEX style emulateapj v. 6/22/04 HYDRODYNAMICS OF COMETARY COMPACT HII REGIONS S. Jane Arthur1 Centro de Radioastronom´ıa y Astrof´ısica, UNAM, Campus Morelia, Apartado Postal 3-72, 58090 Morelia, M´exico and M. G. Hoare School of Physics and Astronomy, University of Leeds, LS2 9JT, UK Draft version November 9, 2018 ABSTRACT We present numerical radiation-hydrodynamic simulations of cometary H II regions for a number of cham- pagne flow and bowshock models. For the champagne flow models we study smooth density distributions with both steep and shallow gradients. We also consider cases where the ionizing star has a strong stellar wind, and cases in which the star additionally has a proper motion within the ambient density gradient. We find that our champagne flow plus stellar wind models have limb-brightenedmorphologiesand kinematics which can see the line-of-sight velocities change sign twice between the head and tail of the cometary H II region, with respect to the rest frame velocity. Our bowshock models show that pressure gradients across and within the shell are very important for the dynamics, and that simple analytic models assuming thin shells in ram pressure balance are wholly inadequate for describing the shape and kinematics of these objects at early times in their evolution. The dynamics of the gas behind the shock in the neutral material ahead of the ionization front in both champagne flow and bowshock type cometary H II regions is also discussed. We present simulated emission-measure maps and long-slit spectra of our results. -
An Ir Study of the Velocity Structure of the Cometary Compact Hii Region
View metadata, citationAn and IR similar study papers ofat core.ac.uk the velocity structure of the cometary compact H II regionbrought to you by CORE provided by CERN Document Server G29.96-0.02 Stuart L. Lumsden Anglo-Australian Observatory, PO Box 296, Epping NSW 2121, Australia and Melvin G. Hoare Max-Planck-Institut f¨ur Astronomie, K¨onigstuhl 17, D-69117, Heidelberg, Germany. ABSTRACT We have mapped the velocity structure of the cometary compact H II region G29.96−0.02 using long-slit echelle spectra of the Brγ line. This technique detects line emission over a much wider area at the necessary spatial resolution compared to radio recombination line observations. Significant structure in both the velocity centroids and the line widths is seen over the entire nebula. Large line widths are seen ahead of the bow and in the tail which may be due to turbulent motions in shocked and interface regions respectively. We construct analytic models of the density and velocity structure in order to attempt to distinguish between the bow shock and champagne flow models which have been put forward to explain the cometary morphology of many compact H II regions. The bow shock model is unable to explain the large velocity gradient that we see right across the tail of the cometary region which can only be explained by the streaming motions towards low density regions in the champagne model. However, our approximation to the champagne model is also not able to fit all of the features of the data. More realistic versions of this model which include the effects of stellar winds and density gradients may be able to provide a better match to these data. -
Radial Velocities of Visual Binaries E
No. 18-September 1979 Radial Velocities of Visual Binaries E. L. van DesseI to the primary. The standard equipment has always been In addition to the initial chemical composition, the a filar micrometer attached to a long-focus refractor. The mass of a star is a fundamental parameter; it tradition is still being kept up, e. g. in Nice (Couteau, Muller, determines the life of the star from its birth, with two refractors of 74 cm und 50 cm aperture-the 74 cm has a focal length of 18 m), Washington (Worley, through its long orshort period ofluminous glory Walker-65 cm refractor), Sproul (Heintz-60 cm). Pairs and to its inevitable end as a compact object. And separated by as little as 0:'10 have been measured. yet, few stars have actually had their mass accu The lOS catalogue ofvisual double stars contained some rately measured. The programme that has re 64,000 stars when it was ed ited in 1963 by Jeffers and van cently been undertaken by Dr. Edwin van DesseI den Bos; the discovery of visual pairs goes steadily on. For of the Royal Observatory in Brussels, Belgium, only about 800, however, enough observations have been collected to determine orbital elements. is therefore of particular importance. By obtain As is weil known, visual binaries are the only means of ing precise measurements of radial velocities obtaining stellar masses, through Kepler's third law. The of stars in double or multiple systems, Dr. mass of a star being one of its basic parameters, it is clear van DesseI expects soon to add new, well that we would like to have as many of them determined as accurately as possible. -
THE STAR FORMATION NEWSLETTER an Electronic Publication Dedicated to Early Stellar Evolution and Molecular Clouds
THE STAR FORMATION NEWSLETTER An electronic publication dedicated to early stellar evolution and molecular clouds No. 129 — 6 July 2003 Editor: Bo Reipurth ([email protected]) Abstracts of recently accepted papers Luminosity Functions of Young Clusters: Modeling the Substellar Mass Regime P.R. Allen1, D.E. Trilling1, D.W. Koerner2, and I.N. Reid3 1 University of Pennsylvania, 209 South 33rd Street, Philadelphia, PA 19104 2 Northern Arizona University, Dept. of Physics and Astronomy, PO Box 6010, Flagstaff, AZ 86011-6010, USA 3 Space Telescope Science Institute, 3700 San Martin Drive, Baltimore, MD 21218, USA E-mail contact: [email protected] We predict near-infrared luminosity functions of young (5 Myr to 1 Gyr) star clusters by combining evolutionary models of very low-mass (1 MJ to 0.15 M ) dwarfs with empirical bolometric corrections. We identify several characteristic features in our results. These can be attributed to three causes: (1) deuterium burning in the most massive substellar objects; (2) methane absorption in bodies with Teff less than 1300 K, the temperature of the L/T transition; and (3) the formation of dust clouds and the rainout of dust at roughly the same effective temperature as methane formation. Accurate reconstruction of the substellar mass function from luminosity function observations requires that these phenomena are taken into account. At present, few observational studies extend to sufficient sensitivities to allow detection of these effects. However, the luminosity function of the young open cluster IC 2391 shows a clear peak at MI ∼ 14 which we attribute to the result of deuterium burning in substellar objects.