Crotalus Oreganus)
Total Page:16
File Type:pdf, Size:1020Kb
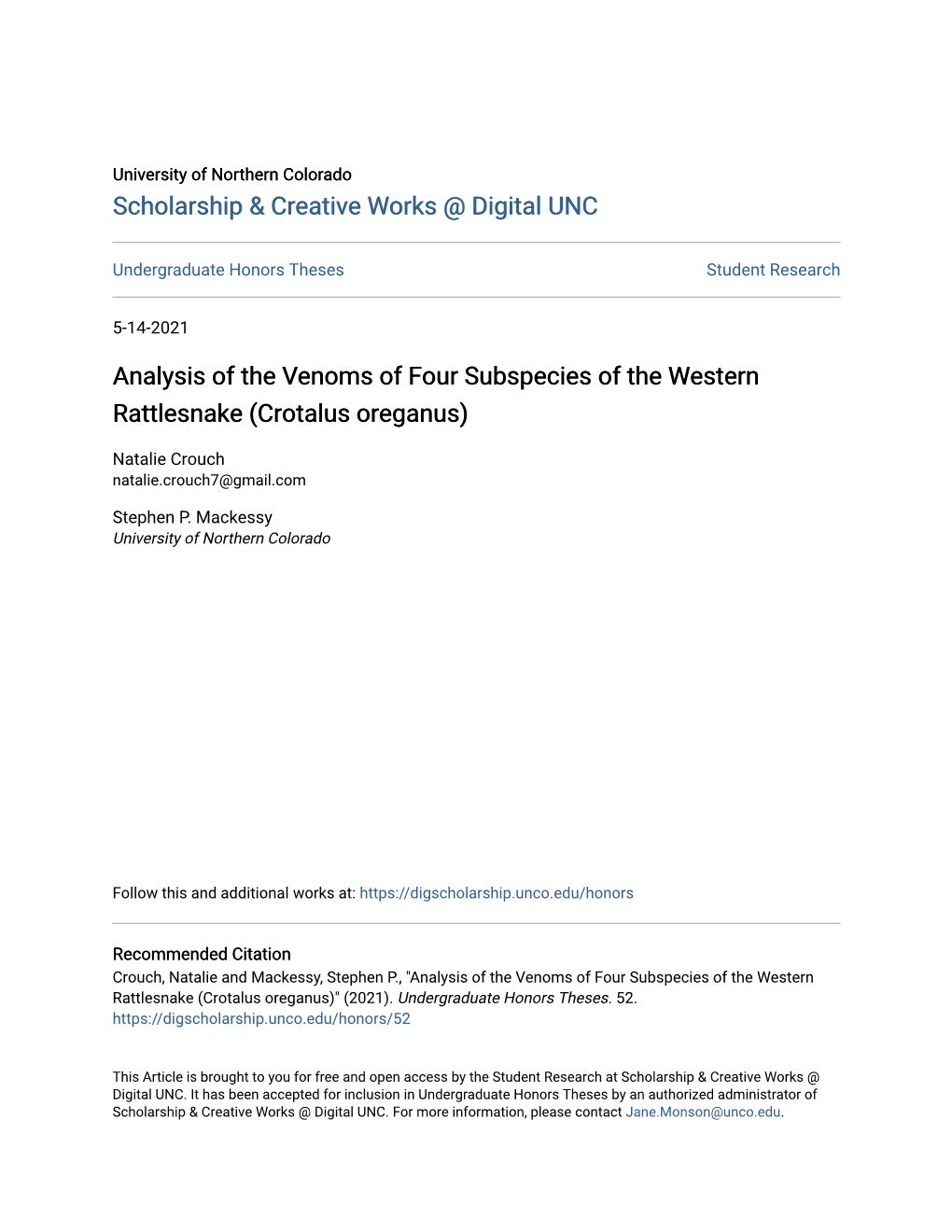
Load more
Recommended publications
-
Species Assessment for the Midget Faded Rattlesnake (Crotalus Viridis Concolor)
SPECIES ASSESSMENT FOR THE MIDGET FADED RATTLESNAKE (CROTALUS VIRIDIS CONCOLOR ) IN WYOMING prepared by 1 2 AMBER TRAVSKY AND DR. GARY P. BEAUVAIS 1 Real West Natural Resource Consulting, 1116 Albin Street, Laramie, WY 82072; (307) 742-3506 2 Director, Wyoming Natural Diversity Database, University of Wyoming, Dept. 3381, 1000 E. University Ave., Laramie, WY 82071; (307) 766-3023 prepared for United States Department of the Interior Bureau of Land Management Wyoming State Office Cheyenne, Wyoming October 2004 Travsky and Beauvais – Crotalus viridus concolor October 2004 Table of Contents INTRODUCTION ................................................................................................................................. 2 NATURAL HISTORY ........................................................................................................................... 2 Morphological Description........................................................................................................... 3 Taxonomy and Distribution ......................................................................................................... 4 Habitat Requirements ................................................................................................................. 6 General ............................................................................................................................................6 Area Requirements..........................................................................................................................7 -
Crotalus Oreganus Concolor: Envenomation Case with Venom Analysis and a Diagnostic Conundrum of Myoneurologic Symptoms
WILDERNESS & ENVIRONMENTAL MEDICINE XXXX; XXX(XXX): 1e6 CASE REPORT Crotalus oreganus concolor: Envenomation Case with Venom Analysis and a Diagnostic Conundrum of Myoneurologic Symptoms Daniel E. Keyler, PharmD 1; Vinay Saini, MD 2;MarkO’Shea, Prof. 3;JeffGee,EMT4; Cara F. Smith, MS 5; Stephen P. Mackessy, PhD 5 1Department of Experimental & Clinical Pharmacology, University of Minnesota, Minneapolis, MN; 2Mimbres Memorial Hospital, Deming, New Mexico; 3Faculty of Science and Engineering, University of Wolverhampton, Wolverhampton, UK; 4Portal Rescue, Portal, AZ; 5School of Biological Sciences, University of Northern Colorado, Greeley, CO A case of midget-faded rattlesnake (Crotalus oreganus concolor) envenomation of an adult male profes- sional herpetologist occurred in a rural setting and resulted in an array of venom induced myoneurologic symptoms. The patient experienced blurry vision, total body paresthesia, dyspnea, chest tightness, and waves of spastic muscle movements of the hands and feet that resembled tetany. It was not apparent whether these symptoms were potentially venom induced or were related to stress-induced physiologic responses. Local envenomation effects were minimal, and coagulation parameters remained within normal limits. Anti- venom was not administered per patient concerns related to a history of acute allergic reactions to antivenom. Venom was collected from the Crotalus oreganus concolor responsible for the bite, and analysis revealed the presence of high levels of myotoxins (SR calcium pump antagonists) and -
Venomous Snakes
Wildlife Damage Management Series Venomous Snakes USU Extension in cooperation with: Gerald W. Wiscomb and Terry A. Messmer Quinney Professorship for Wildlife Conflict Management CNR—Quinney Professorship for Wildlife Conflict Management Utah State University Extension Service and College of Natural Jack H. Berryman Institute Resources Utah Division of Wildlife Resources Department of Fisheries and Wildlife Utah Department of Agriculture and Food Jack H. Berryman Institute USDA/APHIS Animal Damage Control Utah State University, Logan, Utah August 1998 NR/WD/008 Utah is home to 31 species of snakes. Of these, and eyes. (See Figure 1.) These Utah venomous snake seven are venomous. The seven Utah venomous snakes are species include: the sidewinder (Crotalus cerastes), members of the Viperidae family and are commonly called speckled rattlesnake (C. mitchellii), mojave rattlesnake (C. pit vipers because of the pit located between their nostrils scutulatus), western rattlesnake (C. viridis), Hopi Figure 1. Poisonous snakes have vertically elliptical pupils (cat’s eyes), facial pits between the nostril and eye. Non-poisonous snakes have round eye pupils and no facial pits between the nostril and eye. 1 rattlesnake (C.V. nuntius), midget-faded rattlesnake (C.V. Snakes have forked tongues which contain concolor), and the Great Basin rattlesnake (C.V. lutosus). receptors similar to taste buds. They use their tongues to Since most snakes in Utah are non-venomous, most sample odors in the air. Snakes can also use their tongue to snake encounters are generally not dangerous. However, an “sense” their way in the dark as well as locate prey. Pit encounter with a venomous snake can be very dangerous. -
Crotalus Oreganus Concolor: Envenomation Case with Venom Analysis, and a Diagnostic Conundrum of Myo-Neurological Symptoms
1 Crotalus oreganus concolor: Envenomation Case with Venom Analysis, and a Diagnostic Conundrum of Myo-neurological Symptoms Short Title: Crotalus oreganus concolor Envenomation Daniel E. Keyler, PharmD; Vinay Saini, MD; Mark O’Shea, DSc; Jeff Gee, EMT; Cara F Smith, MS; Stephen P Mackessy, PhD From the Department of Experimental & Clinical Pharmacology, University of Minnesota, Minneapolis, MN (Dr Keyler); Mimbres Memorial Hospital, Deming, New Mexico (Dr Saini); Faculty of Science and Engineering, University of Wolverhampton, UK (Professor O’Shea); Portal Rescue, Portal, AZ (Mr. Gee); School of Biological Sciences, University of Northern Colorado, Greeley, CO (Ms Smith and Dr Mackessy). Corresponding Author: Daniel E. Keyler Professor Department of Experimental & Clinical Pharmacology 7-115 Weaver-Densford Hall 308 Harvard St. S.E. University of Minnesota Minneapolis, Minnesota, 55455 USA Phone (612) 840-0425 Email: [email protected] Word Count Abstract - 161 Manuscript + References = 2312 + 723; Total 3035 Reference Count - 33 Figure Count – 3 Table Count - 2 Meeting Presentation Platform presentation by Daniel E Keyler at the Venom Week VI Meeting, March 14, 2018; Texas A&M University, Kingsville, Texas, USA 2 Abstract A case of Midget-faded Rattlesnake (Crotalus oreganus concolor) envenoming to an adult male professional herpetologist occurred in a rural setting and resulted in what appeared as an array of venom induced myo-neurological symptoms. The patient experienced blurry vision, total body paresthesia, dyspnea, chest tightness, and waves of spastic muscle movements of the hands and feet that appeared like tetany. These symptoms were confounding as to whether they were potentially venom induced or were related to stress-induced physiological responses. -
Patterns in Protein Components Present in Rattlesnake Venom: a Meta-Analysis
Preprints (www.preprints.org) | NOT PEER-REVIEWED | Posted: 1 September 2020 doi:10.20944/preprints202009.0012.v1 Article Patterns in Protein Components Present in Rattlesnake Venom: A Meta-Analysis Anant Deshwal1*, Phuc Phan2*, Ragupathy Kannan3, Suresh Kumar Thallapuranam2,# 1 Division of Biology, University of Tennessee, Knoxville 2 Department of Chemistry and Biochemistry, University of Arkansas, Fayetteville 3 Department of Biological Sciences, University of Arkansas, Fort Smith, Arkansas # Correspondence: [email protected] * These authors contributed equally to this work Abstract: The specificity and potency of venom components gives them a unique advantage in development of various pharmaceutical drugs. Though venom is a cocktail of proteins rarely is the synergy and association between various venom components studied. Understanding the relationship between various components is critical in medical research. Using meta-analysis, we found underlying patterns and associations in the appearance of the toxin families. For Crotalus, Dis has the most associations with the following toxins: PDE; BPP; CRL; CRiSP; LAAO; SVMP P-I & LAAO; SVMP P-III and LAAO. In Sistrurus venom CTL and NGF had most associations. These associations can be used to predict presence of proteins in novel venom and to understand synergies between venom components for enhanced bioactivity. Using this approach, the need to revisit classification of proteins as major components or minor components is highlighted. The revised classification of venom components needs to be based on ubiquity, bioactivity, number of associations and synergies. The revised classification will help in increased research on venom components such as NGF which have high medical importance. Keywords: Rattlesnake; Crotalus; Sistrurus; Venom; Toxin; Association Key Contribution: This article explores the patterns of appearance of venom components of two rattlesnake genera: Crotalus and Sistrurus to determine the associations between toxin families. -
Management Plan for the Great Basin National Heritage Area Approved April 30, 2013
Management Plan for the Great Basin National Heritage Area Approved April 30, 2013 Prepared by the Great Basin Heritage Area Partnership Baker, Nevada i ii Great Basin National Heritage Area Management Plan September 23, 2011 Plans prepared previously by several National Heritage Areas provided inspiration for the framework and format for the Great Basin National Heritage Area Management Plan. National Park Service staff and documents provided guidance. We gratefully acknowledge these contributions. This Management Plan was made possible through funding provided by the National Park Service, the State of Nevada, the State of Utah and the generosity of local citizens. 2011 Great Basin National Heritage Area Disclaimer Restriction of Liability The Great Basin Heritage Area Partnership (GBHAP) and the authors of this document have made every reasonable effort to insur e accuracy and objectivity in preparing this plan. However, based on limitations of time, funding and references available, the parties involved make no claims, promises or guarantees about the absolute accuracy, completeness, or adequacy of the contents of this document and expressly disclaim liability for errors and omissions in the contents of this plan. No warranty of any kind, implied, expressed or statutory, including but not limited to the warranties of non-infringement of third party rights, title, merchantability, fitness for a particular purpose, is given with respect to the contents of this document or its references. Reference in this document to any specific commercial products, processes, or services, or the use of any trade, firm or corporation name is for the inf ormation and convenience of the public, and does not constitute endorsement, recommendation, or favoring by the GBHAP or the authors. -
PREDATION of the ENDANGERED BLUNT-NOSED LEOPARD LIZARD (GAMBELIA SILA) in the SAN JOAQUIN DESERT of CALIFORNIA Author: David J
PREDATION OF THE ENDANGERED BLUNT-NOSED LEOPARD LIZARD (GAMBELIA SILA) IN THE SAN JOAQUIN DESERT OF CALIFORNIA Author: David J. Germano Source: The Southwestern Naturalist, 63(4) : 276-280 Published By: Southwestern Association of Naturalists URL: https://doi.org/10.1894/0038-4909-63-4-276 BioOne Complete (complete.BioOne.org) is a full-text database of 200 subscribed and open-access titles in the biological, ecological, and environmental sciences published by nonprofit societies, associations, museums, institutions, and presses. Your use of this PDF, the BioOne Complete website, and all posted and associated content indicates your acceptance of BioOne’s Terms of Use, available at www.bioone.org/terms-of-use. Usage of BioOne Complete content is strictly limited to personal, educational, and non-commercial use. Commercial inquiries or rights and permissions requests should be directed to the individual publisher as copyright holder. BioOne sees sustainable scholarly publishing as an inherently collaborative enterprise connecting authors, nonprofit publishers, academic institutions, research libraries, and research funders in the common goal of maximizing access to critical research. Downloaded From: https://bioone.org/journals/The-Southwestern-Naturalist on 22 Oct 2019 Terms of Use: https://bioone.org/terms-of-use Access provided by Southwestern Association of Naturalists THE SOUTHWESTERN NATURALIST 63(4): 276–280 PREDATION OF THE ENDANGERED BLUNT-NOSED LEOPARD LIZARD (GAMBELIA SILA) IN THE SAN JOAQUIN DESERT OF CALIFORNIA DAVID J. GERMANO Department of Biology, California State University, Bakersfield, CA 93311-1099 Correspondent: [email protected] ABSTRACT—Predation can significantly affect prey populations, which could be significant for recovering species threatened with extinction. -
DEPARTMENT of WILDLIFE Wildlife Diversity Division 6980 Sierra Center Parkway, Ste 120 • Reno, Nevada 89511 (775) 688-1500 Fax (775) 688-1987
STATE OF NEVADA DEPARTMENT OF WILDLIFE Wildlife Diversity Division 6980 Sierra Center Parkway, Ste 120 • Reno, Nevada 89511 (775) 688-1500 Fax (775) 688-1987 MEMORANDUM September 1, 2017 To: Nevada Board of Wildlife Commissioners, County Advisory Boards to Manage Wildlife, and Interested Publics From: Jennifer Newmark, Administrator, Wildlife Diversity Division Title: Commercial Collection of Reptiles Description: The Commission will consider at least two potential regulations for commercial collection of reptiles. One regulation could prohibit all collection of reptiles for commercial purposes, either temporarily or permanently. The other regulation could limit collection of reptiles for commercial purposes based on season, species, collection area and/or take. Options will be discussed and the Commission may choose to direct the Department to advance a recommendation to a future Commission meeting. Summary: At the August 2017 meeting, the Commission directed the Department to draft two potential regulations for the Commission to discuss regarding the commercial collection of reptiles. The following is a summary of options the Department has drafted that could be included in future regulations, policies, or directions that the Commission may choose to take. A Commission General Regulation (CGR) would need to be drafted if the Commission chose to prohibit commercial collection of reptiles, but if the Commission chose to limit commercial collection of reptiles based on season, species, collection area and/or take a Commission Regulation (CR) would need to be drafted. Information on both options is provided below. A Possible Commission General Regulation (CGR) to Prohibit Commercial Collection of Reptiles This regulation would prohibit collection of reptiles for commercial purposes in the State of Nevada, either temporarily or permanently. -
(Crotalus Oreganus Helleri) Hunting Behavior Through Community Science
diversity Article Quantifying Southern Pacific Rattlesnake (Crotalus oreganus helleri) Hunting Behavior through Community Science Emily R. Urquidi * and Breanna J. Putman Department of Biology, California State University San Bernardino, 5500 University Parkway, San Bernardino, CA 92407, USA; [email protected] * Correspondence: [email protected] Abstract: It is increasingly important to study animal behaviors as these are the first responses organisms mount against environmental changes. Rattlesnakes, in particular, are threatened by habitat loss and human activity, and require costly tracking by researchers to quantify the behaviors of wild individuals. Here, we show how photo-vouchered observations submitted by community members can be used to study cryptic predators like rattlesnakes. We utilized two platforms, iNaturalist and HerpMapper, to study the hunting behaviors of wild Southern Pacific Rattlesnakes. From 220 observation photos, we quantified the direction of the hunting coil (i.e., “handedness”), microhabitat use, timing of observations, and age of the snake. With these data, we looked at whether snakes exhibited an ontogenetic shift in behaviors. We found no age differences in coil direction. However, there was a difference in the microhabitats used by juveniles and adults while hunting. We also found that juveniles were most commonly observed during the spring, while adults were more consistently observed throughout the year. Overall, our study shows the potential of using Citation: Urquidi, E.R.; Putman, B.J. community science to study the behaviors of cryptic predators. Quantifying Southern Pacific Rattlesnake (Crotalus oreganus helleri) Keywords: citizen science; conservation; ontogeny; behavioral lateralization; snakes Hunting Behavior through Community Science. Diversity 2021, 13, 349. https://doi.org/10.3390/ d13080349 1. -
List1 Stránka 1 Agama Agama Agama Agama Agama
List1 Adax Adax nasomaculatus Agama Acanthosaura capra Agama Physignathus lesueri Agama Schinisaurus crocodilurus Agama Pogona vitticeps Agama Hydrosaurs pustulatus Agama hardůn Stellio stellio Agama himalájská Stellio himalayanus Agama kavkazská Stellio caucasicus Agama lehmanova Stellio lehmanni Agama límcová Chlamydosaurus kingii Agama motýlí Leiolepis belliana Agama mozambická Agama mossambica Agama stepní Tropelus sanquinolentus Agama vodní Physignathus cocincinus Agama webrova Hydrosaurus weberi Agamka písečná Phrynocephalus mystaceus Agamka sluneční Phrynocephalus helioscopus persicus Aguti zlatý Dasyprocta aguti Akuči červený Myoprocta acouchy Alexandr čínský Psittacula derbiana Alexandr malý Psittacula krameri Aligátor čínský Alligator sinensis Aligátor severoamerický Alligator mississippiensis Amazoňan červenočelý menší Amazona d.rhodocorytha Amazoňan červenočelý větší Amazona d.dufresiana Amazoňan fialovotemenný menší Amazona f.finschi Amazoňan haitský Amazona ventralis Amazoňan jamajský Amazona colaria Amazoňan kubánský Amazona leucocephala Amazoňan modrobradý Amazona festiva Amazoňan modročelý Amazona aestiva Amazoňan modročelý větší Amazona aestiva xanthopteryx Amazoňan oranžovokřídlý Amazona amazonica Amazoňan pomoučený karibský Amazona farinosa guatemalae Amazoňan vějířový Deroptyus accipitrinus Amazoňan vínorudý Amazona vinacea Amazoňan žlutohlavý Amazona ochrocephala Amazoňan žlutohlavý velký Amazona ochrocephala oratrix Amazoňan žlutolící ekvádorský Amazona autumnalis lilacina Amazoňan žlutolící větší Amazona autumnalis -
Venomous Reptiles of Nevada
Venomous Reptiles of Nevada Figure 1 The buzz from a rattlesnake can signal a heart stopping adventure to even the most experienced outdoor enthusiast. Figure 2 Authors M. L. Robinson, Area Specialist, Water/Environmental Horticulture, University of Nevada Cooperative Extension Polly M. Conrad, Wildlife Diversity Biologist—Reptiles, Nevada Department of Wildlife Maria M. Ryan, Area Specialist, Natural Resources, University of Nevada Cooperative Extension Updated from G. Mitchell, M.L. Robinson, D.B. Hardenbrook and E.L. Sellars. 1998. What’s the Buzz About Nevada’s Venomous Reptiles? University of Nevada Cooperative Extension—Nevada Department of Wildlife Partnership Publication. FS-98-35. SP 07-07 (Replaces FS-98-35) NEVADA’S REPTILES Approximately 52 species of snakes and lizards share the Nevada landscape with us. Of these, only 12 are considered venomous. Only 6 can be dangerous to people and pets. Encountering them is uncommon because of their body camouflage and secretive nature, which are their first defenses in evading predators. Consider yourself fortunate if you do see one! As with all wildlife, treat venomous reptiles with respect. Reptiles are ectothermic, meaning their body temperature increases or decreases in response to the surrounding environment. They are most active in the spring, summer and early fall when it’s comfortable, short sleeve weather for us. Reptiles usually hibernate, or brumate, in winter in response to colder temperatures. During high summer temperatures in the Mojave Desert, reptiles may spend time underground in order to maintain vital body temperatures. In most cases*, collecting Nevada’s native reptiles is not allowed without the appropriate permit, which is issued by the Nevada Department of Wildlife. -
Effects of Disturbance on the Northern Pacific Rattlesnake (Crotalus Oreganus Oreganus) in British Columbia
EFFECTS OF DISTURBANCE ON THE NORTHERN PACIFIC RATTLESNAKE (CROTALUS OREGANUS OREGANUS) IN BRITISH COLUMBIA by EMILY V. LOMAS BSc., University of British Columbia, 2009 A THESIS SUBMITTED IN PARTIAL FULFILLMENT OF THE REQUIREMENTS FOR THE DEGREE OF Master of Science in Environmental Science Thompson Rivers University Kamloops, British Columbia, Canada April 2013 Thesis examining committee: Karl Larsen (PhD), Professor and Thesis Supervisor, Natural Resource Sciences, Thompson Rivers University Lauchlan Fraser (PhD), Professor, Natural Resource Sciences, Thompson Rivers University Robert Hood (PhD), Associate Professor, Tourism Management Department, Thompson Rivers University Christine Bishop (PhD), Research Scientist, Environment Canada Leigh Anne Isaac (PhD) (External Examiner), Wildlife Biologist, VAST Resource Solutions Inc. ii ACKNOWLEDGEMENTS I would like to sincerely thank my supervisor, Dr. Karl Larsen, for presenting me with the opportunity to work with, arguably, one of Canada’s most unique and extraordinary animals. His patience, supportive work environment, and first-rate stories have been greatly appreciated. Special thanks to Dr. Christine Bishop, without whom this project would not have been possible. Dr. Bishop’s dedication to the snake research program and support of my research has been invaluable. My experience working with snakes has been fascinating, challenging, and one I will never forget. Thanks also to my committee members Dr. Lauchlan Fraser and Dr. Robert Hood for their time, insight, and feedback. My time at Nk’Mip Desert Cultural Centre has been one full of new friends and fond memories. A huge thank you to Charlotte Stringam, who was very supportive and always made me feel more than welcome. Margaret Holm was instrumental in securing funds for the project and helping me with the small details with always speedy responses.