1 September 12, 2014
Total Page:16
File Type:pdf, Size:1020Kb
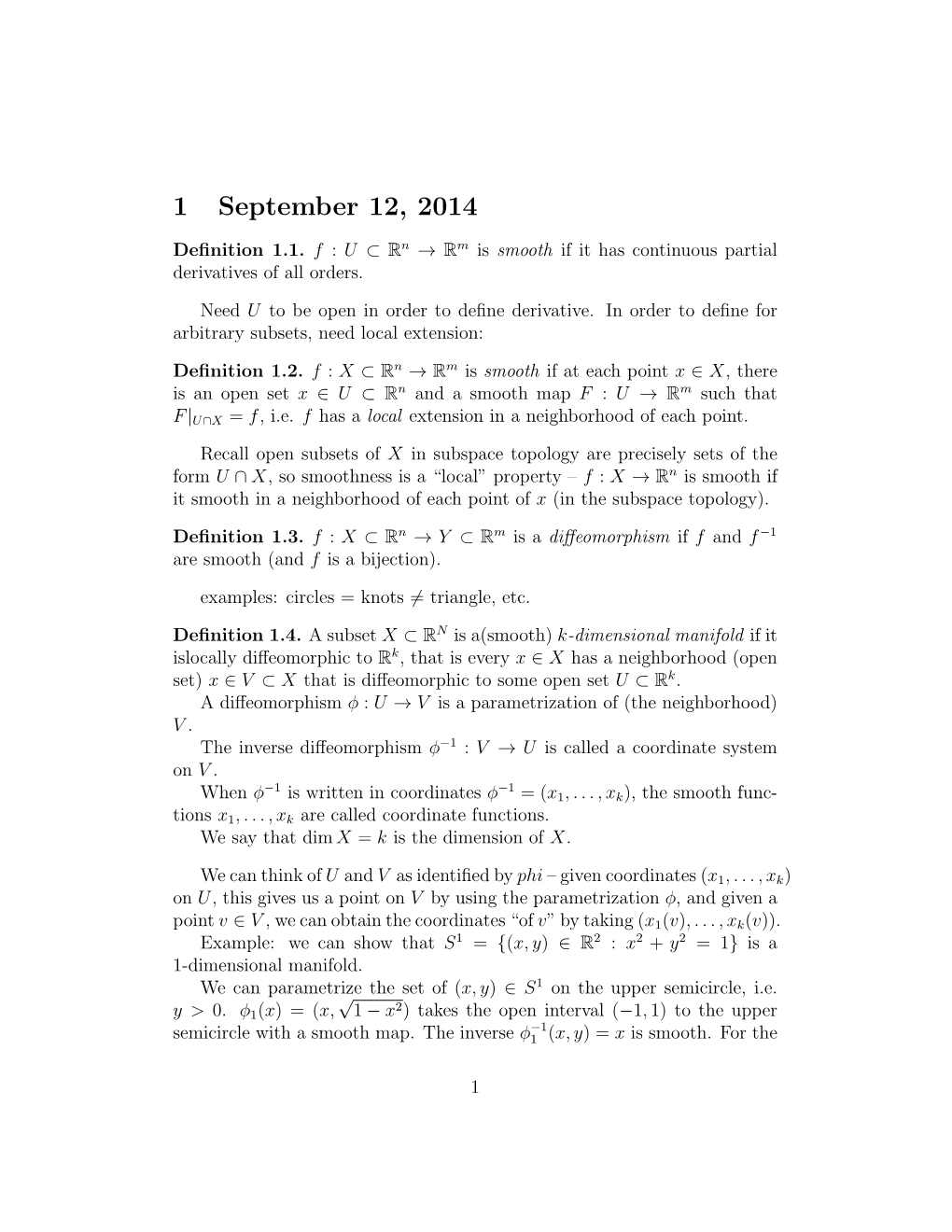
Load more
Recommended publications
-
Codimension Zero Laminations Are Inverse Limits 3
CODIMENSION ZERO LAMINATIONS ARE INVERSE LIMITS ALVARO´ LOZANO ROJO Abstract. The aim of the paper is to investigate the relation between inverse limit of branched manifolds and codimension zero laminations. We give necessary and sufficient conditions for such an inverse limit to be a lamination. We also show that codimension zero laminations are inverse limits of branched manifolds. The inverse limit structure allows us to show that equicontinuous codimension zero laminations preserves a distance function on transver- sals. 1. Introduction Consider the circle S1 = { z ∈ C | |z| = 1 } and the cover of degree 2 of it 2 p2(z)= z . Define the inverse limit 1 1 2 S2 = lim(S ,p2)= (zk) ∈ k≥0 S zk = zk−1 . ←− Q This space has a natural foliated structure given by the flow Φt(zk) = 2πit/2k (e zk). The set X = { (zk) ∈ S2 | z0 = 1 } is a complete transversal for the flow homeomorphic to the Cantor set. This space is called solenoid. 1 This construction can be generalized replacing S and p2 by a sequence of compact p-manifolds and submersions between them. The spaces obtained this way are compact laminations with 0 dimensional transversals. This construction appears naturally in the study of dynamical systems. In [17, 18] R.F. Williams proves that an expanding attractor of a diffeomor- phism of a manifold is homeomorphic to the inverse limit f f f S ←− S ←− S ←−· · · where f is a surjective immersion of a branched manifold S on itself. A branched manifold is, roughly speaking, a CW-complex with tangent space arXiv:1204.6439v2 [math.DS] 20 Nov 2012 at each point. -
Planar Embeddings of Minc's Continuum and Generalizations
PLANAR EMBEDDINGS OF MINC’S CONTINUUM AND GENERALIZATIONS ANA ANUSIˇ C´ Abstract. We show that if f : I → I is piecewise monotone, post-critically finite, x X I,f and locally eventually onto, then for every point ∈ =←− lim( ) there exists a planar embedding of X such that x is accessible. In particular, every point x in Minc’s continuum XM from [11, Question 19 p. 335] can be embedded accessibly. All constructed embeddings are thin, i.e., can be covered by an arbitrary small chain of open sets which are connected in the plane. 1. Introduction The main motivation for this study is the following long-standing open problem: Problem (Nadler and Quinn 1972 [20, p. 229] and [21]). Let X be a chainable contin- uum, and x ∈ X. Is there a planar embedding of X such that x is accessible? The importance of this problem is illustrated by the fact that it appears at three independent places in the collection of open problems in Continuum Theory published in 2018 [10, see Question 1, Question 49, and Question 51]. We will give a positive answer to the Nadler-Quinn problem for every point in a wide class of chainable continua, which includes←− lim(I, f) for a simplicial locally eventually onto map f, and in particular continuum XM introduced by Piotr Minc in [11, Question 19 p. 335]. Continuum XM was suspected to have a point which is inaccessible in every planar embedding of XM . A continuum is a non-empty, compact, connected, metric space, and it is chainable if arXiv:2010.02969v1 [math.GN] 6 Oct 2020 it can be represented as an inverse limit with bonding maps fi : I → I, i ∈ N, which can be assumed to be onto and piecewise linear. -
A Geometric Take on Metric Learning
A Geometric take on Metric Learning Søren Hauberg Oren Freifeld Michael J. Black MPI for Intelligent Systems Brown University MPI for Intelligent Systems Tubingen,¨ Germany Providence, US Tubingen,¨ Germany [email protected] [email protected] [email protected] Abstract Multi-metric learning techniques learn local metric tensors in different parts of a feature space. With such an approach, even simple classifiers can be competitive with the state-of-the-art because the distance measure locally adapts to the struc- ture of the data. The learned distance measure is, however, non-metric, which has prevented multi-metric learning from generalizing to tasks such as dimensional- ity reduction and regression in a principled way. We prove that, with appropriate changes, multi-metric learning corresponds to learning the structure of a Rieman- nian manifold. We then show that this structure gives us a principled way to perform dimensionality reduction and regression according to the learned metrics. Algorithmically, we provide the first practical algorithm for computing geodesics according to the learned metrics, as well as algorithms for computing exponential and logarithmic maps on the Riemannian manifold. Together, these tools let many Euclidean algorithms take advantage of multi-metric learning. We illustrate the approach on regression and dimensionality reduction tasks that involve predicting measurements of the human body from shape data. 1 Learning and Computing Distances Statistics relies on measuring distances. When the Euclidean metric is insufficient, as is the case in many real problems, standard methods break down. This is a key motivation behind metric learning, which strives to learn good distance measures from data. -
Inverse Vs Implicit Function Theorems - MATH 402/502 - Spring 2015 April 24, 2015 Instructor: C
Inverse vs Implicit function theorems - MATH 402/502 - Spring 2015 April 24, 2015 Instructor: C. Pereyra Prof. Blair stated and proved the Inverse Function Theorem for you on Tuesday April 21st. On Thursday April 23rd, my task was to state the Implicit Function Theorem and deduce it from the Inverse Function Theorem. I left my notes at home precisely when I needed them most. This note will complement my lecture. As it turns out these two theorems are equivalent in the sense that one could have chosen to prove the Implicit Function Theorem and deduce the Inverse Function Theorem from it. I showed you how to do that and I gave you some ideas how to do it the other way around. Inverse Funtion Theorem The inverse function theorem gives conditions on a differentiable function so that locally near a base point we can guarantee the existence of an inverse function that is differentiable at the image of the base point, furthermore we have a formula for this derivative: the derivative of the function at the image of the base point is the reciprocal of the derivative of the function at the base point. (See Tao's Section 6.7.) Theorem 0.1 (Inverse Funtion Theorem). Let E be an open subset of Rn, and let n f : E ! R be a continuously differentiable function on E. Assume x0 2 E (the 0 n n base point) and f (x0): R ! R is invertible. Then there exists an open set U ⊂ E n containing x0, and an open set V ⊂ R containing f(x0) (the image of the base point), such that f is a bijection from U to V . -
Part III : the Differential
77 Part III : The differential 1 Submersions In this section we will introduce topological and PL submersions and we will prove that each closed submersion with compact fibres is a locally trivial fibra- tion. We will use Γ to stand for either Top or PL and we will suppose that we are in the category of Γ–manifolds without boundary. 1.1 A Γ–map p: Ek → Xl betweenΓ–manifoldsisaΓ–submersion if p is locally the projection Rk−→πl R l on the first l–coordinates. More precisely, p: E → X is a Γ–submersion if there exists a commutative diagram p / E / X O O φy φx Uy Ux ∩ ∩ πl / Rk / Rl k l where x = p(y), Uy and Ux are open sets in R and R respectively and ϕy , ϕx are charts around x and y respectively. It follows from the definition that, for each x ∈ X ,thefibre p−1(x)isaΓ– manifold. 1.2 The link between the notion of submersions and that of bundles is very straightforward. A Γ–map p: E → X is a trivial Γ–bundle if there exists a Γ– manifold Y and a Γ–isomorphism f : Y × X → E , such that pf = π2 ,where π2 is the projection on X . More generally, p: E → X is a locally trivial Γ–bundle if each point x ∈ X has an open neighbourhood restricted to which p is a trivial Γ–bundle. Even more generally, p: E → X is a Γ–submersion if each point y of E has an open neighbourhood A, such that p(A)isopeninXand the restriction A → p(A) is a trivial Γ–bundle. -
Neural Subgraph Matching
Neural Subgraph Matching NEURAL SUBGRAPH MATCHING Rex Ying, Andrew Wang, Jiaxuan You, Chengtao Wen, Arquimedes Canedo, Jure Leskovec Stanford University and Siemens Corporate Technology ABSTRACT Subgraph matching is the problem of determining the presence of a given query graph in a large target graph. Despite being an NP-complete problem, the subgraph matching problem is crucial in domains ranging from network science and database systems to biochemistry and cognitive science. However, existing techniques based on combinatorial matching and integer programming cannot handle matching problems with both large target and query graphs. Here we propose NeuroMatch, an accurate, efficient, and robust neural approach to subgraph matching. NeuroMatch decomposes query and target graphs into small subgraphs and embeds them using graph neural networks. Trained to capture geometric constraints corresponding to subgraph relations, NeuroMatch then efficiently performs subgraph matching directly in the embedding space. Experiments demonstrate that NeuroMatch is 100x faster than existing combinatorial approaches and 18% more accurate than existing approximate subgraph matching methods. 1.I NTRODUCTION Given a query graph, the problem of subgraph isomorphism matching is to determine if a query graph is isomorphic to a subgraph of a large target graph. If the graphs include node and edge features, both the topology as well as the features should be matched. Subgraph matching is a crucial problem in many biology, social network and knowledge graph applications (Gentner, 1983; Raymond et al., 2002; Yang & Sze, 2007; Dai et al., 2019). For example, in social networks and biomedical network science, researchers investigate important subgraphs by counting them in a given network (Alon et al., 2008). -
DIFFERENTIAL GEOMETRY Contents 1. Introduction 2 2. Differentiation 3
DIFFERENTIAL GEOMETRY FINNUR LARUSSON´ Lecture notes for an honours course at the University of Adelaide Contents 1. Introduction 2 2. Differentiation 3 2.1. Review of the basics 3 2.2. The inverse function theorem 4 3. Smooth manifolds 7 3.1. Charts and atlases 7 3.2. Submanifolds and embeddings 8 3.3. Partitions of unity and Whitney’s embedding theorem 10 4. Tangent spaces 11 4.1. Germs, derivations, and equivalence classes of paths 11 4.2. The derivative of a smooth map 14 5. Differential forms and integration on manifolds 16 5.1. Introduction 16 5.2. A little multilinear algebra 17 5.3. Differential forms and the exterior derivative 18 5.4. Integration of differential forms on oriented manifolds 20 6. Stokes’ theorem 22 6.1. Manifolds with boundary 22 6.2. Statement and proof of Stokes’ theorem 24 6.3. Topological applications of Stokes’ theorem 26 7. Cohomology 28 7.1. De Rham cohomology 28 7.2. Cohomology calculations 30 7.3. Cechˇ cohomology and de Rham’s theorem 34 8. Exercises 36 9. References 42 Last change: 26 September 2008. These notes were originally written in 2007. They have been classroom-tested twice. Address: School of Mathematical Sciences, University of Adelaide, Adelaide SA 5005, Australia. E-mail address: [email protected] Copyright c Finnur L´arusson 2007. 1 1. Introduction The goal of this course is to acquire familiarity with the concept of a smooth manifold. Roughly speaking, a smooth manifold is a space on which we can do calculus. Manifolds arise in various areas of mathematics; they have a rich and deep theory with many applications, for example in physics. -
LECTURE 6: FIBER BUNDLES in This Section We Will Introduce The
LECTURE 6: FIBER BUNDLES In this section we will introduce the interesting class of fibrations given by fiber bundles. Fiber bundles play an important role in many geometric contexts. For example, the Grassmaniann varieties and certain fiber bundles associated to Stiefel varieties are central in the classification of vector bundles over (nice) spaces. The fact that fiber bundles are examples of Serre fibrations follows from Theorem ?? which states that being a Serre fibration is a local property. 1. Fiber bundles and principal bundles Definition 6.1. A fiber bundle with fiber F is a map p: E ! X with the following property: every ∼ −1 point x 2 X has a neighborhood U ⊆ X for which there is a homeomorphism φU : U × F = p (U) such that the following diagram commutes in which π1 : U × F ! U is the projection on the first factor: φ U × F U / p−1(U) ∼= π1 p * U t Remark 6.2. The projection X × F ! X is an example of a fiber bundle: it is called the trivial bundle over X with fiber F . By definition, a fiber bundle is a map which is `locally' homeomorphic to a trivial bundle. The homeomorphism φU in the definition is a local trivialization of the bundle, or a trivialization over U. Let us begin with an interesting subclass. A fiber bundle whose fiber F is a discrete space is (by definition) a covering projection (with fiber F ). For example, the exponential map R ! S1 is a covering projection with fiber Z. Suppose X is a space which is path-connected and locally simply connected (in fact, the weaker condition of being semi-locally simply connected would be enough for the following construction). -
TOPOLOGY HW 8 55.1 Show That If a Is a Retract of B 2, Then Every
TOPOLOGY HW 8 CLAY SHONKWILER 55.1 Show that if A is a retract of B2, then every continuous map f : A → A has a fixed point. 2 Proof. Suppose r : B → A is a retraction. Thenr|A is the identity map on A. Let f : A → A be continuous and let i : A → B2 be the inclusion map. Then, since i, r and f are continuous, so is F = i ◦ f ◦ r. Furthermore, by the Brouwer fixed-point theorem, F has a fixed point x0. In other words, 2 x0 = F (x0) = (i ◦ f ◦ r)(x0) = i(f(r(x0))) ∈ A ⊆ B . Since x0 ∈ A, r(x0) = x0 and so x0F (x0) = i(f(r(x0))) = i(f(x0)) = f(x0) since i(x) = x for all x ∈ A. Hence, x0 is a fixed point of f. 55.4 Suppose that you are given the fact that for each n, there is no retraction f : Bn+1 → Sn. Prove the following: (a) The identity map i : Sn → Sn is not nulhomotopic. Proof. Suppose i is nulhomotopic. Let H : Sn × I → Sn be a homotopy between i and a constant map. Let π : Sn × I → Bn+1 be the map π(x, t) = (1 − t)x. π is certainly continuous and closed. To see that it is surjective, let x ∈ Bn+1. Then x x π , 1 − ||x|| = (1 − (1 − ||x||)) = x. ||x|| ||x|| Hence, since it is continuous, closed and surjective, π is a quotient map that collapses Sn×1 to 0 and is otherwise injective. Since H is constant on Sn×1, it induces, by the quotient map π, a continuous map k : Bn+1 → Sn that is an extension of i. -
Quantum Query Complexity and Distributed Computing ILLC Dissertation Series DS-2004-01
Quantum Query Complexity and Distributed Computing ILLC Dissertation Series DS-2004-01 For further information about ILLC-publications, please contact Institute for Logic, Language and Computation Universiteit van Amsterdam Plantage Muidergracht 24 1018 TV Amsterdam phone: +31-20-525 6051 fax: +31-20-525 5206 e-mail: [email protected] homepage: http://www.illc.uva.nl/ Quantum Query Complexity and Distributed Computing Academisch Proefschrift ter verkrijging van de graad van doctor aan de Universiteit van Amsterdam op gezag van de Rector Magnificus prof.mr. P.F. van der Heijden ten overstaan van een door het college voor promoties ingestelde commissie, in het openbaar te verdedigen in de Aula der Universiteit op dinsdag 27 januari 2004, te 12.00 uur door Hein Philipp R¨ohrig geboren te Frankfurt am Main, Duitsland. Promotores: Prof.dr. H.M. Buhrman Prof.dr.ir. P.M.B. Vit´anyi Overige leden: Prof.dr. R.H. Dijkgraaf Prof.dr. L. Fortnow Prof.dr. R.D. Gill Dr. S. Massar Dr. L. Torenvliet Dr. R.M. de Wolf Faculteit der Natuurwetenschappen, Wiskunde en Informatica The investigations were supported by the Netherlands Organization for Sci- entific Research (NWO) project “Quantum Computing” (project number 612.15.001), by the EU fifth framework projects QAIP, IST-1999-11234, and RESQ, IST-2001-37559, the NoE QUIPROCONE, IST-1999-29064, and the ESF QiT Programme. Copyright c 2003 by Hein P. R¨ohrig Revision 411 ISBN: 3–933966–04–3 v Contents Acknowledgments xi Publications xiii 1 Introduction 1 1.1 Computation is physical . 1 1.2 Quantum mechanics . 2 1.2.1 States . -
MTH 304: General Topology Semester 2, 2017-2018
MTH 304: General Topology Semester 2, 2017-2018 Dr. Prahlad Vaidyanathan Contents I. Continuous Functions3 1. First Definitions................................3 2. Open Sets...................................4 3. Continuity by Open Sets...........................6 II. Topological Spaces8 1. Definition and Examples...........................8 2. Metric Spaces................................. 11 3. Basis for a topology.............................. 16 4. The Product Topology on X × Y ...................... 18 Q 5. The Product Topology on Xα ....................... 20 6. Closed Sets.................................. 22 7. Continuous Functions............................. 27 8. The Quotient Topology............................ 30 III.Properties of Topological Spaces 36 1. The Hausdorff property............................ 36 2. Connectedness................................. 37 3. Path Connectedness............................. 41 4. Local Connectedness............................. 44 5. Compactness................................. 46 6. Compact Subsets of Rn ............................ 50 7. Continuous Functions on Compact Sets................... 52 8. Compactness in Metric Spaces........................ 56 9. Local Compactness.............................. 59 IV.Separation Axioms 62 1. Regular Spaces................................ 62 2. Normal Spaces................................ 64 3. Tietze's extension Theorem......................... 67 4. Urysohn Metrization Theorem........................ 71 5. Imbedding of Manifolds.......................... -
Tight Immersions of Simplicial Surfaces in Three Space
View metadata, citation and similar papers at core.ac.uk brought to you by CORE provided by Elsevier - Publisher Connector Pergamon Topology Vol. 35, No. 4, pp. 863-873, 19% Copyright Q 1996 Elsevier Sctence Ltd Printed in Great Britain. All tights reserved CO4&9383/96 $15.00 + 0.00 0040-9383(95)00047-X TIGHT IMMERSIONS OF SIMPLICIAL SURFACES IN THREE SPACE DAVIDE P. CERVONE (Received for publication 13 July 1995) 1. INTRODUCTION IN 1960,Kuiper [12,13] initiated the study of tight immersions of surfaces in three space, and showed that every compact surface admits a tight immersion except for the Klein bottle, the real projective plane, and possibly the real projective plane with one handle. The fate of the latter surface went unresolved for 30 years until Haab recently proved [9] that there is no smooth tight immersion of the projective plane with one handle. In light of this, a recently described simplicial tight immersion of this surface [6] came as quite a surprise, and its discovery completes Kuiper’s initial survey. Pinkall [17] broadened the question in 1985 by looking for tight immersions in each equivalence class of immersed surfaces under image homotopy. He first produced a complete description of these classes, and proceeded to generate tight examples in all but finitely many of them. In this paper, we improve Pinkall’s result by giving tight simplicial examples in all but two of the immersion classes under image homotopy for which tight immersions are possible, and in particular, we point out and resolve an error in one of his examples that would have left an infinite number of immersion classes with no tight examples.