Dendritic Spines and Development: Towards a Unifying Model of Spinogenesis—A Present Day Review of Cajal’S Histological Slides and Drawings
Total Page:16
File Type:pdf, Size:1020Kb
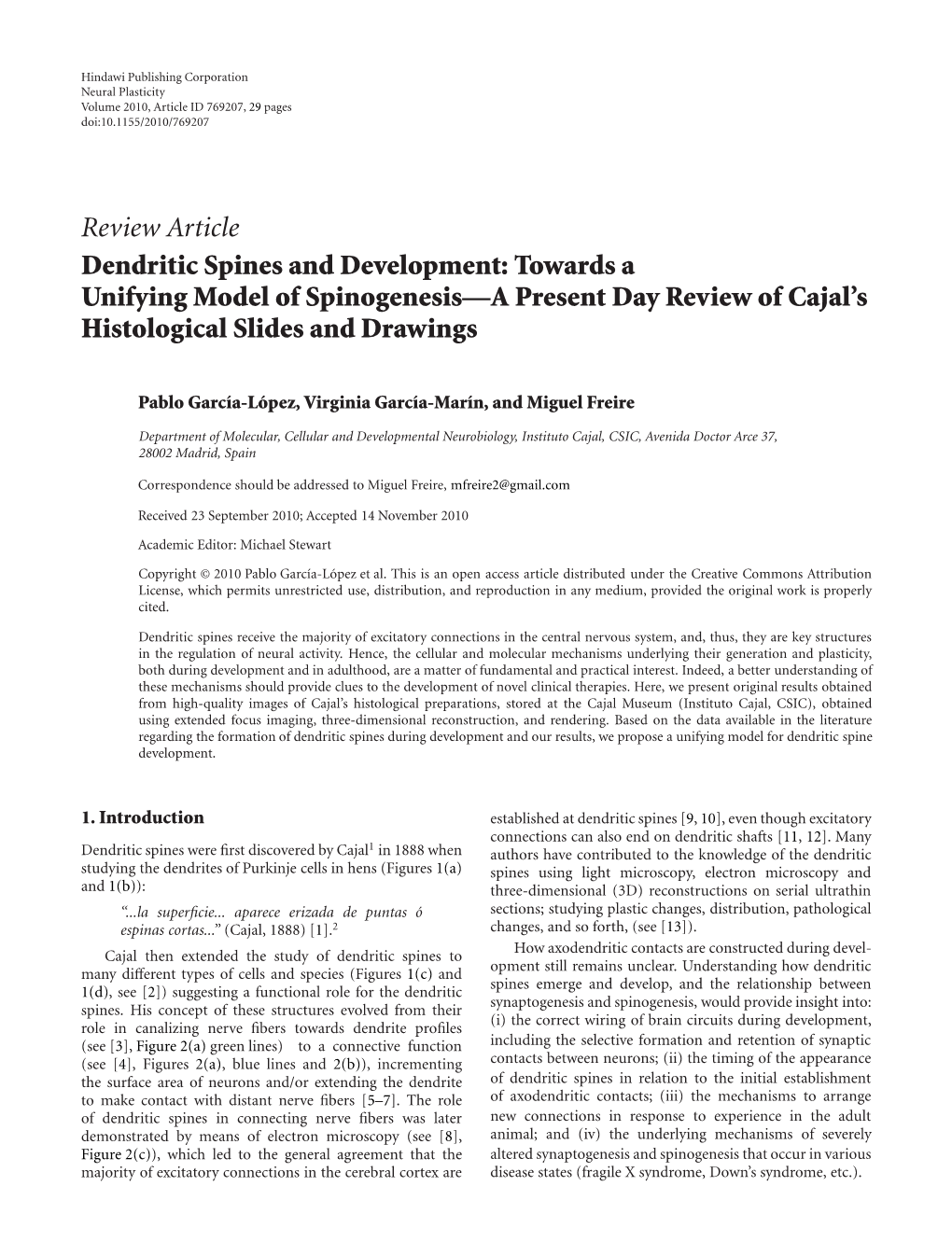
Load more
Recommended publications
-
Early Postnatal Development of Pyramidal Neurons Across Layers Of
www.nature.com/scientificreports OPEN Early postnatal development of pyramidal neurons across layers of the mouse medial prefrontal cortex Received: 20 November 2018 Tim Kroon 1,2, Eline van Hugte1,3, Lola van Linge1,4, Huibert D. Mansvelder1 & Accepted: 12 March 2019 Rhiannon M. Meredith1 Published: xx xx xxxx Mammalian neocortex is a highly layered structure. Each layer is populated by distinct subtypes of principal cells that are born at diferent times during development. While the diferences between principal cells across layers have been extensively studied, it is not known how the developmental profles of neurons in diferent layers compare. Here, we provide a detailed morphological and functional characterisation of pyramidal neurons in mouse mPFC during the frst postnatal month, corresponding to known critical periods for synapse and neuron formation in mouse sensory neocortex. Our data demonstrate similar maturation profles of dendritic morphology and intrinsic properties of pyramidal neurons in both deep and superfcial layers. In contrast, the balance of synaptic excitation and inhibition difers in a layer-specifc pattern from one to four postnatal weeks of age. Our characterisation of the early development and maturation of pyramidal neurons in mouse mPFC not only demonstrates a comparable time course of postnatal maturation to that in other neocortical circuits, but also implies that consideration of layer- and time-specifc changes in pyramidal neurons may be relevant for studies in mouse models of neuropsychiatric and neurodevelopmental disorders. Pyramidal neurons (PNs) in diferent cortical layers difer in their expression of molecular markers1–3, responses to sensory stimuli4, patterns of synaptic connectivity5, and morphological properties6–9. -
Perturbations of Dendritic Excitability in Epilepsy
Jasper's Basic Mechanisms of the Epilepsies Jasper's Basic Mechanisms of the Epilepsies Perturbations of Dendritic Excitability in Epilepsy Cha-Min Tang1 Scott M. Thompson2 1 Departments of Neurology and Physiology, University of Maryland School of Medicine; Baltimore VA, Medical Center 2 Department of Physiology, University of Maryland School of Medicine Abstract The dendritic arbor is the site of complex interactions between synaptic excitation and intrinsic excitability. It has become apparent recently, that the dendritic arbor cannot be viewed as a passive antenna that simply receives and relays synaptic input to the cell body. Instead, dendrites express an abundance of voltage-gated channels that are capable of initiating regenerative spikes and actively regulate the local dendritic resting membrane potential. Active properties can be expressed as back- propagating action potentials along the main apical trunk and as localized spikes confined to individual terminal dendritic segments. The notion of the dendritic arbor as a highly active structure has profound implications for the generation of epilepsy. This chapter will focus on recent data on perturbations to dendritic intrinsic excitability associated with epileptic conditions. An attempt will be made to understand how hyperexcitability may be the result of maladaptive homeostatic mechanism. Topics to be addressed relevant to the functional reorganization of dendrites include activity-dependent down regulation of IA (Kv4.2), epilepsy induced down regulation of Ih (HCN1 and HCN2), and deafferentation induced down regulation of SK-type potassium channels. Other topics to be addressed include the concept of electrical compartmentalization within the dendritic arbor and the recruitment of NMDA receptors as part of intrinsic excitability. -
Action Potentials in Basal and Oblique Dendrites of Rat Neocortical Pyramidal Neurons Srdjan D
Physiology in Press; published online on May 9, 2003 as 10.1113/jphysiol.2002.033746 J Physiol (2003), xxx.x, pp. 000–000 DOI: 10.1113/jphysiol.2002.033746 © The Physiological Society 2003 www.jphysiol.org Action potentials in basal and oblique dendrites of rat neocortical pyramidal neurons Srdjan D. Antic Department of Cellular and Molecular Physiology, and Department of Neurobiology, Yale University School of Medicine, 333 Cedar Street, New Haven, CT 06520, USA Basal and oblique dendrites comprise ~2/3 of the total excitable membrane in the mammalian cerebral cortex, yet they have never been probed with glass electrodes, and therefore their electrical properties and overall impact on synaptic processing are unknown. In the present study, fast multi- site voltage-sensitive dye imaging combined with somatic recording was used to provide a detailed description of the membrane potential transients in basal and oblique dendrites of pyramidal neurons during single and trains of action potentials (APs). The optical method allowed simultaneous measurements from several dendrites in the visual field up to 200 mm from the soma, thus providing a unique report on how an AP invades the entire dendritic tree. In contrast to apical dendrites, basal and oblique branches: (1) impose very little amplitude and time course modulation on backpropagating APs; (2) are strongly invaded by the somatic spike even when somatic firing rates reach 40 Hz (activity-independent backpropagation); and (3) do not exhibit signs of a ‘calcium shoulder’ on the falling phase of the AP. A compartmental model incorporating AP peak latencies and half-widths obtained from the apical, oblique and basal dendrites indicates that the specific intracellular resistance (Ri) is less than 100 V cm. -
Geometry and the Organizational Principle of Spine Synapses Along a Dendrite
Research Article: New Research | Neuronal Excitability Geometry and the organizational principle of spine synapses along a dendrite https://doi.org/10.1523/ENEURO.0248-20.2020 Cite as: eNeuro 2020; 10.1523/ENEURO.0248-20.2020 Received: 8 June 2020 Revised: 2 October 2020 Accepted: 7 October 2020 This Early Release article has been peer-reviewed and accepted, but has not been through the composition and copyediting processes. The final version may differ slightly in style or formatting and will contain links to any extended data. Alerts: Sign up at www.eneuro.org/alerts to receive customized email alerts when the fully formatted version of this article is published. Copyright © 2020 Parajuli et al. This is an open-access article distributed under the terms of the Creative Commons Attribution 4.0 International license, which permits unrestricted use, distribution and reproduction in any medium provided that the original work is properly attributed. 1 1. Manuscript Title (11 words): Geometry and the organizational principle of spine 2 synapses along a dendrite 3 2. Abbreviated title (46 characters): Synapse organizational principle in a dendrite 4 3. Authors name and affiliation: 5 Laxmi Kumar Parajuli1,2, Hidetoshi Urakubo3, Ai Takahashi-Nakazato1, Roberto Ogelman4, 6 Hirohide Iwasaki1,5, Masato Koike2, Hyung-Bae Kwon6,7, Shin Ishii3, Won Chan Oh4,6, Yugo 7 Fukazawa8, Shigeo Okabe1 8 1Department of Cellular Neurobiology, Graduate School of Medicine, The University of 9 Tokyo, Tokyo 113-0033, Japan. 2Department of Cell Biology and Neuroscience, Juntendo 10 University Graduate School of Medicine, Tokyo 113-8421, Japan. 3Department of Systems 11 Science, Graduate School of Informatics, Kyoto University, Kyoto 606-8501, Japan. -
Pneumonia-Induced Endothelial Amyloids Reduce Dendritic Spine Density in Brain Neurons Allison M
www.nature.com/scientificreports OPEN Pneumonia-induced endothelial amyloids reduce dendritic spine density in brain neurons Allison M. Scott1, Alexandrea C. Jager1, Meredith Gwin1,4, Sarah Voth1,4, Ron Balczon2,4, Troy Stevens1,3,4 & Mike T. Lin1,4 ✉ Pseudomonas aeruginosa pneumonia elicits endothelial cell release of cytotoxic amyloids that can be recovered from the bronchoalveolar lavage and cerebrospinal fuids of critically ill patients. Introduction of these cytotoxic amyloids into the lateral ventricle impairs learning and memory in mice. However, it is unclear whether the amyloids of lung origin (1) are neurotropic, and (2) cause structural remodeling of hippocampal dendrites. Thus, we used electrophysiological studies in brain slices and structural analysis of post-mortem tissues obtained from animals exposed to endothelium-derived amyloids to assess these issues. The amyloids were administered via three diferent routes, by intracerebroventricular, intratracheal, and intraperitoneal injections. Synaptic long-term potentiation was abolished following intracerebroventricular amyloid injection. Fluorescence dialysis or Golgi-impregnation labeling showed reduced dendritic spine density and destabilized spines of hippocampal pyramidal neurons 4 weeks after intracerebroventricular amyloid injection. In comparison, endothelial amyloids introduced to the airway caused the most prominent dendritic spine density reduction, yet intraperitoneal injection of these amyloids did not afect spine density. Our fndings indicate that infection-elicited lung endothelial amyloids are neurotropic and reduce neuronal dendritic spine density in vivo. Amyloids applied into the trachea may either be disseminated through the circulation and cross the blood-brain barrier to access the brain, initiate feed-forward amyloid transmissibility among cells of the blood-brain barrier or access the brain in other ways. -
Abnormal Excitability of Oblique Dendrites Implicated in Early Alzheimer’S: a Computational Study
ORIGINAL RESEARCH ARTICLE published: 31 May 2010 NEURAL CIRCUITS doi: 10.3389/fncir.2010.00016 Abnormal excitability of oblique dendrites implicated in early Alzheimer’s: a computational study Thomas M. Morse1*, Nicholas T. Carnevale1, Pradeep G. Mutalik 2, Michele Migliore1,3 and Gordon M. Shepherd 1 1 Department of Neurobiology, Yale University School of Medicine, New Haven, CT, USA 2 Center for Medical Informatics, Yale University School of Medicine, New Haven, CT, USA 3 Institute of Biophysics, National Research Council, Palermo, Italy Edited by: The integrative properties of cortical pyramidal dendrites are essential to the neural basis of Nelson Spruston, Northwestern cognitive function, but the impact of amyloid beta protein (aβ) on these properties in early University, USA Alzheimer’s is poorly understood. In animal models, electrophysiological studies of proximal Reviewed by: + Dalton J. Surmeier, Northwestern dendrites have shown that aβ induces hyperexcitability by blocking A-type K currents (IA), University, USA disrupting signal integration. The present study uses a computational approach to analyze the Nelson Spruston, Northwestern hyperexcitability induced in distal dendrites beyond the experimental recording sites. The results University, USA show that back-propagating action potentials in the dendrites induce hyperexcitability and *Correspondence: excessive calcium concentrations not only in the main apical trunk of pyramidal cell dendrites, Thomas M. Morse, Department of Neurobiology, Yale University School of but also in their oblique dendrites. Evidence is provided that these thin branches are particularly Medicine, P.O. Box 208001, sensitive to local reductions in IA. The results suggest the hypothesis that the oblique branches New Haven, CT 06520-8001, USA. -
Preferential Localization of Muscarinic M1 Receptor on Dendritic Shaft and Spine of Cortical Pyramidal Cells and Its Anatomical Evidence for Volume Transmission
4408 • The Journal of Neuroscience, March 24, 2010 • 30(12):4408–4418 Cellular/Molecular Preferential Localization of Muscarinic M1 Receptor on Dendritic Shaft and Spine of Cortical Pyramidal Cells and Its Anatomical Evidence for Volume Transmission Miwako Yamasaki,1 Minoru Matsui,2 and Masahiko Watanabe1,3 1Department of Anatomy, Hokkaido University Graduate School of Medicine, Sapporo 060-8638, Japan, 2Department of Clinical Research and General Medicine, Tokyo-Nishi Tokushukai Hospital, Tokyo, 196-0003, Japan, and 3Japan Science and Technology Agency, Core Research for Evolutional Science and Technology (CREST), Sanbocho, Chiyada-ku, Tokyo 102-0075, Japan Acetylcholine (ACh) plays important roles for higher brain functions, including arousal, attention, and cognition. These effects are mediated largely by muscarinic acetylcholine receptors (mAChRs). However, it remains inconclusive whether the mode of ACh-mAChR signaling is synaptic, so-called “wired,” transmission mediated by ACh released into the synaptic cleft, or nonsynaptic, so-called “vol- ume,” transmission by ambient ACh. To address this issue, we examined cellular and subcellular distribution of M1 , the most predom- inant mAChR subtype in the cerebral cortex and hippocampus, and pursued its anatomical relationship with cholinergic varicosities in these regions of adult mice. M1 was highly expressed in glutamatergic pyramidal neurons, whereas it was low or undetectable in various GABAergic interneuron subtypes. M1 was preferentially distributed on the extrasynaptic membrane of pyramidal cell dendrites and spines. Cholinergic varicosities often made direct contact to pyramidal cell dendrites and synapses. At such contact sites, however, synapse-like specialization was infrequent, and no particular accumulation was found at around contact sites for both M1 and presyn- patic active zone protein Bassoon. -
Review Article Dendritic Spines and Development: Towards a Unifying Model of Spinogenesis—A Present Day Review of Cajal’S Histological Slides and Drawings
Hindawi Publishing Corporation Neural Plasticity Volume 2010, Article ID 769207, 29 pages doi:10.1155/2010/769207 Review Article Dendritic Spines and Development: Towards a Unifying Model of Spinogenesis—A Present Day Review of Cajal’s Histological Slides and Drawings Pablo Garcıa-L´ opez,´ Virginia Garcıa-Mar´ ın,´ and Miguel Freire Department of Molecular, Cellular and Developmental Neurobiology, Instituto Cajal, CSIC, Avenida Doctor Arce 37, 28002 Madrid, Spain Correspondence should be addressed to Miguel Freire, [email protected] Received 23 September 2010; Accepted 14 November 2010 Academic Editor: Michael Stewart Copyright © 2010 Pablo Garcıa-L´ opez´ et al. This is an open access article distributed under the Creative Commons Attribution License, which permits unrestricted use, distribution, and reproduction in any medium, provided the original work is properly cited. Dendritic spines receive the majority of excitatory connections in the central nervous system, and, thus, they are key structures in the regulation of neural activity. Hence, the cellular and molecular mechanisms underlying their generation and plasticity, both during development and in adulthood, are a matter of fundamental and practical interest. Indeed, a better understanding of these mechanisms should provide clues to the development of novel clinical therapies. Here, we present original results obtained from high-quality images of Cajal’s histological preparations, stored at the Cajal Museum (Instituto Cajal, CSIC), obtained using extended focus imaging, three-dimensional reconstruction, and rendering. Based on the data available in the literature regarding the formation of dendritic spines during development and our results, we propose a unifying model for dendritic spine development. 1. Introduction established at dendritic spines [9, 10], even though excitatory connections can also end on dendritic shafts [11, 12]. -
Biphasic Dendritic Growth of Dorsolateral Prefrontal Cortex Associative Neurons and Early Cognitive Development
BASIC SCIENCE 189 Croat Med J. 2018;59:189-202 https://doi.org/10.3325/cmj.2018.59.189 Biphasic dendritic growth of Dora Sedmak1,2, Branka Hrvoj-Mihić3, Domagoj dorsolateral prefrontal cortex Džaja1,2, Nikola Habek2,4, Harry B. M. Uylings5, associative neurons and early Zdravko Petanjek1,2 cognitive development 1Department of Anatomy and Clinical Anatomy, University of Zagreb School of Medicine, Zagreb, Croatia 2Croatian Institute for Brain Research, University of Zagreb School of Medicine, Zagreb, Croatia Aim To analyze postnatal development and life-span 3Department of Anthropology, changes of apical dendrite side branches (oblique den- University of California San Diego, drites) from associative layer IIIC magnopyramidal neurons La Jolla, CA, USA in the human dorsolateral prefrontal cortex and to com- 4Department of Physiology and pare the findings with the previously established pattern Immunology, University of Zagreb of basal dendrite development. School of Medicine, Zagreb, Croatia 5Department of Anatomy and Methods We analyzed dendritic morphology from 352 Neuroscience, Graduate School rapid-Golgi impregnated neurons (10-18 neurons per sub- of Neurosciences Amsterdam, ject) in Brodmann area 9 from the post-mortem tissue of Amsterdam University Medical 25 subjects ranging in age from 1 week to 91 years. Data Center, Amsterdam, the were collected in the period between 1994 and 1996, and Netherlands the analysis was performed between September 2017 and *DS and BHM contributed equally February 2018. Quantitative dendritic parameters were sta- tistically analyzed using one-way analysis of variance and two-tailed t tests. Results Oblique dendrites grew rapidly during the first postnatal months, and the increase in the dendrite length was accompanied by the outgrowth of new dendritic seg- ments. -
Somogyi 2010 Hippocampus- Intrinsic Organisation.Pdf
1 Hippocampus – intrinsic organisation subm: 29. 7. 2009 In: Handbook of Brain Microcircuits, Oxford University Press Eds: Gordon M. Shepherd, Sten Grillner Peter Somogyi MRC Anatomical Neuropharmacology Unit, Department of Pharmacology, University of Oxford, Oxford OX1 3TH, UK. Tel: 44 1865 271 898 Fax: 44 1865 271 648 e-mail: [email protected] Figures: 3 Table: 1 The hippocampus (CA1, CA2 and CA3 areas and the dentate gyrus) together with the subiculum represents an associational area of the cerebral cortex intimately involved in mnemonic processes. Through its connections with other areas of the temporal lobe, the hippocampus contributes to the encoding, association, consolidation and recall of representations of the external and internal world in the combined firing rates and spike timing of glutamatergic pyramidal and granule cells. The hippocampus is thought to associate specific life events (items, episodes), on several time scales, in temporally determined firing sequences of neuronal assemblies (see chapter by Buzsaki). A single pyramidal cell can be part of several cell assemblies with different partners and contribute to different representations. Pyramidal cell assemblies are thought to be kept together and segregated from other assemblies by the dynamic strengthening and weakening of glutamatergic synaptic weights as well as by GABAergic interneurons. Interneurons generate cell domain and brain state dependent rhythmic changes in excitability, which are key for the formation, consolidation and recall of representations. Unsurprisingly, interneurons show intricate spatio-temporal diversity; e.g. the CA1 area is served by at least 21 types of resident GABAergic cell. I will attempt to allocate explicit roles for some of them, based on their previously published firing patterns in vivo as observed in identified neurons recorded in anaesthetised rats and on their putative equivalents in non-anaesthetised animals (Freund and Buzsaki, 1996; Somogyi and Klausberger, 2005; Klausberger and Somogyi, 2008). -
Cortical Influences on Cognitive and Respiratory
CORTICAL INFLUENCES ON COGNITIVE AND RESPIRATORY DYSFUNCTION IN A MOUSE MODEL OF RETT SYNDROME By CODY JAMES HOWELL Submitted in partial fulfillment of the requirements for the degree of Doctor of Philosophy Dissertation Advisor: David M. Katz Ph.D. Department of Neurosciences CASE WESTERN RESERVE UNIVERSITY May 2019 Case Western Reserve University School of Graduate Studies We hereby approve the dissertation of Cody James Howell candidate for the degree of Doctor of Philosophy Committee Chair............................................................ Heather T. Broihier, Ph.D. Committee Member........................................................... David M. Katz, Ph.D. Committee Member..................................................... Evan S. Deneris, Ph.D. Committee Member............................................................. Thomas E. Dick, Ph.D. February 12th 2019 *We also certify that written approval has been obtained for any proprietary material contained therein 2 Contents List of Figures ……………......................................................................................7 List of Tables .......................................................................................................10 Abstract................................................................................................................11 Preface…………………………....…………………………………………………….13 Chapter 1: Introduction.....................................................................................16 Rett Syndrome: Clinical Presentation..................................................................16 -
Synaptic Input Sequence Discrimination on Behavioral Timescales Mediated by Reaction-Diffusion Chemistry in Dendrites Upinder Singh Bhalla*
RESEARCH ARTICLE Synaptic input sequence discrimination on behavioral timescales mediated by reaction-diffusion chemistry in dendrites Upinder Singh Bhalla* National Centre for Biological Sciences, Tata Institute of Fundamental Research, Bangalore, India Abstract Sequences of events are ubiquitous in sensory, motor, and cognitive function. Key computational operations, including pattern recognition, event prediction, and plasticity, involve neural discrimination of spatio-temporal sequences. Here, we show that synaptically-driven reaction-diffusion pathways on dendrites can perform sequence discrimination on behaviorally relevant time-scales. We used abstract signaling models to show that selectivity arises when inputs at successive locations are aligned with, and amplified by, propagating chemical waves triggered by previous inputs. We incorporated biological detail using sequential synaptic input onto spines in morphologically, electrically, and chemically detailed pyramidal neuronal models based on rat data. Again, sequences were recognized, and local channel modulation downstream of putative sequence-triggered signaling could elicit changes in neuronal firing. We predict that dendritic sequence-recognition zones occupy 5 to 30 microns and recognize time-intervals of 0.2 to 5 s. We suggest that this mechanism provides highly parallel and selective neural computation in a functionally important time range. DOI: 10.7554/eLife.25827.001 Introduction *For correspondence: bhalla@ Activity sequences have long been recognized as a fundamental constituent of neural processing. ncbs.res.in Lorente de No suggested that reverberatory activity sequences in small networks could sustain activ- Competing interest: See ity (Lorente de No, 1938). Hebb’s idea of cell assemblies suggested that ensembles of cells page 21 encoded a particular neuronal concept, but also that there was sequential activation within the group of cells forming the assembly (Hebb, 1949).