Efficacy of Systemic Insecticides Against the Citrus Mealybug
Total Page:16
File Type:pdf, Size:1020Kb
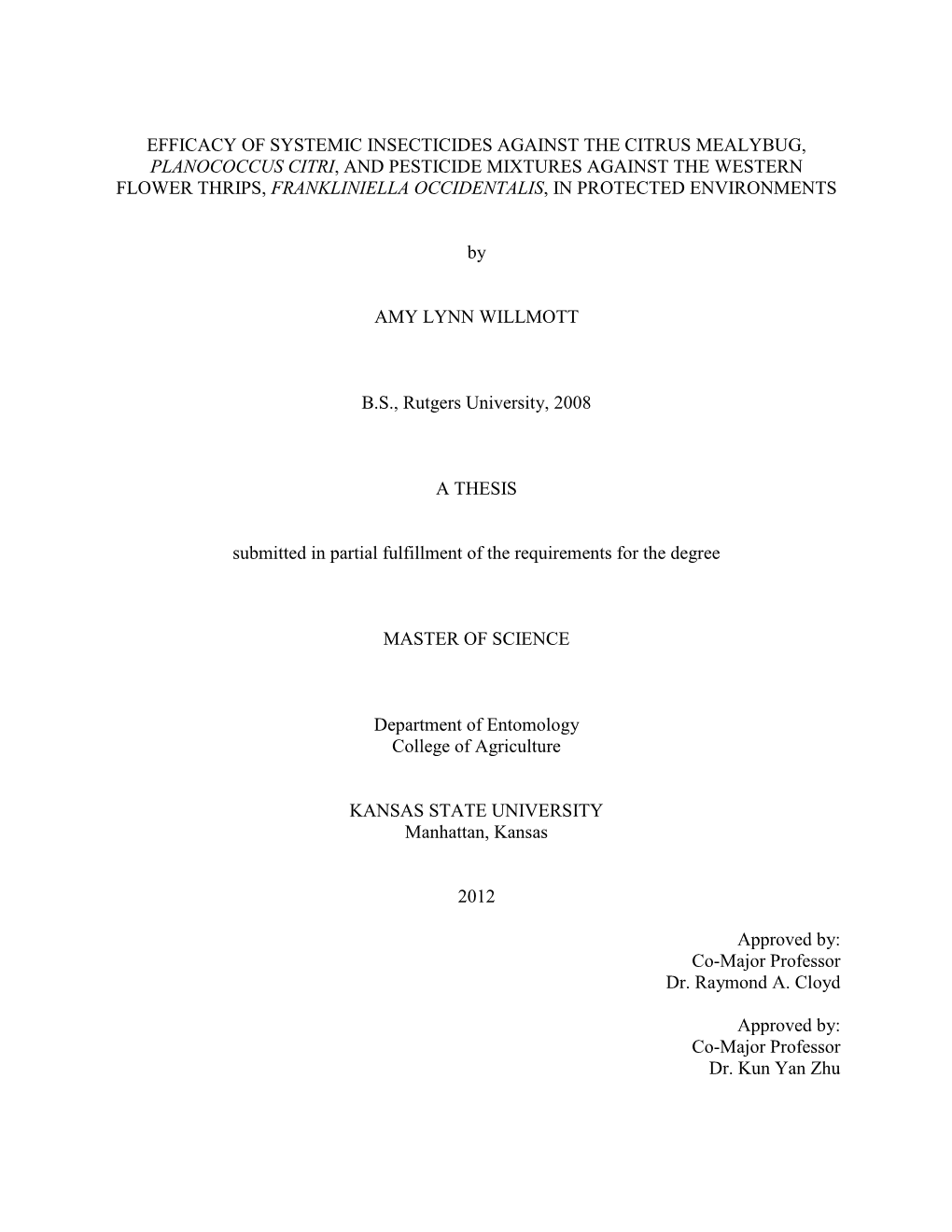
Load more
Recommended publications
-
New Records of Some Pests of the Coconut Inflorescence and Developing Fruit and Their Natural Enemies in Sri Lanka
COCOS, (1987) 5, 39—42 Printed in Sri Lanka New Records of some Pests of the Coconut Inflorescence and Developing Fruit and Their Natural Enemies in Sri Lanka L. C. P. FERNANDO and P. KANAGARATNAM Coconut Research Institute, Lunuwila, Sri Lanka. ABSTRACT A survey was carried out at Bandirippuwa Estate, Kirimetiyana Estate and at Isolated Seed Garden, Rajakadaluwa for the pests of coconut inflorescence and developing fruits. The mite, Dolichotetranychus sp. (Tenuipalpidae) which lives beneath the perianth and feeds on the epicarp, causes considerable damage to the developing fruit. Bi-monthly observations on the intensity of infestation of nuts among different forms of coconut indicated a significant difference among forms in their susceptibility to mite damage. Pseudococcus cocotis (Maskell) (Pseudococcidae), Pseudococcus citriculus Green (Pseudococcidae) and Planococcus lilacinus (Cockereli) (Pseudococcidae), when feeding in large numbers on the peduncle, cause button nut shedding and drying up of the inflo rescence. The parasitoids and predators of these mealybugs recorded for the first time in Sri Lanka are Promuscidea unfasciativentris Girault (Aphelinidae) Platygaster sp. (Platygastridae), Anagyrus sp. nr. pseudococci (Girault) (Encyrtidae) Coccodiplosis sp. (Cecidomyiidae), Cryptogonus bryanti Kapur (Coccinellidae) and Pseudoscymnus sp. (Coccinellidae). Several species of scale insects namely, Coccus hesperidum (Linnaeus) (Coccidae) Aulacaspis sp. (Diaspididae), Pseudaulacaspis cockerelli (Cooley) (Diaspididae), Aoni- diella orientalis (Newstead) (Diaspididae), and Pinnaspis strachani (Cooley) (Diaspididae) are recorded as minor pests of the developing fruits of coconut. Coccophagus silvestrii Compere (Aphelinidae), Scymnus sp. (Coccinellidae), Pseudoscymnus sp. (Coccinellidae) Telsimia ceylonica (Weise) (Coccinellidae), Cryptogonus bryanti Kapur (Coccinellidae) and Cybocephalus sp. (Nitidulidae) are recorded as their parasitoids and predators. These natural enemies are probably responsible for the control of these pests in the field. -
Evaluating the Effect of Some Botanical Insecticides on the Citrus Mealybug Planococcus Citri (Risso) (Hemiptera: Pseudococcidae)
African Journal of Biotechnology Vol. 11(53), pp. 11620-11624, 3 July, 2012 Available online at http://www.academicjournals.org/AJB DOI:10.5897//AJB11.4226 ISSN 1684-5315 ©2012 Academic Journals Full Length Research Paper Evaluating the effect of some botanical insecticides on the citrus mealybug Planococcus citri (Risso) (Hemiptera: Pseudococcidae) Ahmadi, M.1, Amiri-Besheli, B.1* and Hosieni, S. Z.2 1Department of Agricultural Entomology Sari Agricultural University, Sari, Iran. 2Plant Breeding Sari Agricultural University, Sari, Iran. Accepted 23 May, 2012 Planococcus citri (Risso) (Homoptera: Pseudococcidae), is one of the key pests of citrus. The use of chemical pesticides for a long time can cause many problems such as pesticide resistance, as well as having an adverse effect on the environment. The use of chemical pesticides needs to be replaced with non-chemical control methods. The effects of tondexir (pepper extract) and palizin (eucalyptus extract) using five doses (500, 1000, 1500, 2000 and 3000 ppm) and sirinol (garlic extract) with five doses (1000, 1500, 2000, 2500 and 3500 ppm) on citrus mealybug was investigated. The effect of barter (a botanical synergist) using a single dose (1000 ppm) being added to tondexir and palizin at three doses (500, 1000 and 1500 ppm) and barter (1000 ppm) added to sirinol at four doses (1000, 1500, 2000 and 2500) on citrus mealybug was examined. Mortality was recorded after 24, 48, 72 and 96 h post-treatments. Analysis of variance showed that the highest mortality with 3000 ppm doses of tondexir and palizin was 90/60 ± 2/93 and 89/16 ± 1/92% with sirinol (3500 ppm) with 87.11 ± 1.11% mortality, respectively. -
Laboratory Studies on the Development and Life Table Parameters of Planococcus Citri (Risso) (Hemiptera, Pseudococcidae) at Different Temperatures
International Journal of Research Studies in Biosciences (IJRSB) Volume 4, Issue 5, May 2016, PP 16-25 ISSN 2349-0357 (Print) & ISSN 2349-0365 (Online) http://dx.doi.org/10.20431/2349-0365.0405003 www.arcjournals.org Laboratory Studies on the Development and Life Table Parameters of Planococcus citri (Risso) (Hemiptera, Pseudococcidae) at Different Temperatures M. A. El-Aw1, Kh. A. A. Draz1, H. H. Karam2, A. A. A. Khalafallah3 1Plant Protection Department, Faculty of Agriculture, Damanhour University, Egypt 2Department of Applied Entomology, Faculty of Agriculture (El-Shatby), Alexandria University, Egypt 3Plant Protection Research Institute, Agriculture Research Center, Giza, Egypt [email protected] Abstract: The development and life table parameters of Planococcus citri (Risso) were investigated at various temperatures ranging from 20 to 30 ºC. The total duration of development of female mealybug was 28.2 day at 20 ºC, 20.8 day at 25 ºC, and 20.05 day at 30ºC. The total life cycles of P. citri females were 63.20, 51.10, and 41.55 days at 20, 25, and 30ºC, respectively. The highest number of egg laying by female of P. citri was recorded at 30˚C with an average of 340.1 eggs, while the average numbers of egg laying were 62.1 and 276.8 eggs per female at 20, and 25˚C, respectively. The total life cycles of P. citri males were 43.2, 30.4, and 23.95 days at 20, 25, and 30ºC, respectively. The total number of daughters per female (Ro) obtained at 20ºC was 62.61 compared with 136.88, 380.00 and 339.62 daughters at 25, 27 and 30 ºC, respectively. -
Feeding Potential of Cryptolaemus Montrouzieri Against the Mealybug Phenacoccus Solenopsis
Phytoparasitica DOI 10.1007/s12600-011-0211-3 Feeding potential of Cryptolaemus montrouzieri against the mealybug Phenacoccus solenopsis Harmeet Kaur & J. S. Virk Received: 25 August 2011 /Accepted: 1 December 2011 # Springer Science+Business Media B.V. 2011 Abstract Phenacoccus solenopsis Tinsley (Hemiptera: Keywords Biocontrol agent . Cotton . Ladybird . Pseudococcidae) is an exotic species native to the USA, North India damaging cotton and other plant families. The feeding potential of different development stages of Cryptolae- mus montrouzieri Mulsant, a biological control agent Introduction against mealybugs, was investigated on different devel- opment stages of P. solenopsis. Fourth instar grubs and Mealybugs are sap-sucking insects that cause severe adults of C. montrouzieri were the most voracious economic damage to a wide range of crops (Nagrare et feeders on different instars of mealybug. The number al. 2009). The cotton mealybug, Phenacoccus sole- of 1st instar nymphs of mealybug consumed by 1st,2nd, nopsis Tinsley (Hemiptera: Pseudococcidae), was 3rd and 4th instar larvae and adult beetles of C. montrou- reported originally on ornamental and fruit crops in zieri was 15.56, 41.01, 125.38, 162.69 and 1613.81, the United States (Tinsley 1898) and regarded as an respectively. The respective numbers of 2nd and 3rd exotic pest in South East Asia, including India and instar nymphs of mealybug consumed were 11.15 and Pakistan. Fuchs et al. (1991) provided the first report 1.80, 26.35 and 6.36, 73.66 and 13.32, 76.04 and 21.16, of P. solenopsis infesting cultivated cotton and 29 787.95 and 114.66. -
Biology of Planococcus Citri (Risso) (Hemiptera: Pseudococcidae) on Five Yam Varieties in Storage
Advances in Entomology, 2014, 2, 167-175 Published Online October 2014 in SciRes. http://www.scirp.org/journal/ae http://dx.doi.org/10.4236/ae.2014.24025 Biology of Planococcus citri (Risso) (Hemiptera: Pseudococcidae) on Five Yam Varieties in Storage Emmanuel Asiedu, Jakpasu Victor Kofi Afun, Charles Kwoseh Department of Crop and Soil Sciences, College of Agriculture and Natural Resources, Kwame Nkrumah University of Science and Technology, Kumasi, Ghana Email: [email protected] Received 25 June 2014; revised 30 July 2014; accepted 18 August 2014 Copyright © 2014 by authors and Scientific Research Publishing Inc. This work is licensed under the Creative Commons Attribution International License (CC BY). http://creativecommons.org/licenses/by/4.0/ Abstract Yam is an important staple cash crop, which constitutes 53% of total root and tuber consumption in West Africa. It is a cheap source of carbohydrate in the diets of millions of people worldwide and in tropical West Africa. However, attack by Planococcus citri results in shriveling of the tubers, making them become light and unpalatable. They also lose their market value. The total number of eggs laid, incubation period, developmental period and adult longevity of P. citri on stored yam Disocorea species were studied on five yam varieties namely Dioscorea rotundata var. Pona, Dios- corea rotundata var. Labreko, Dioscorea rotundata var. Muchumudu, Disocorea alata var. Matches and Dioscorea rotundata var. Dente in the laboratory with ambient temperatures of 26.0˚C - 30.0˚C and relative humidity of 70.0% - 75.0%. The mean life spans of the female insect that is from hatch to death on Dioscorea rotundata var. -
Arthropod Pest Management in Greenhouses and Interiorscapes E
Arthropod Pest Management in Greenhouses and Interiorscapes E-1011E-1011 OklahomaOklahoma CooperativeCooperative ExtensionExtension ServiceService DivisionDivision ofof AgriculturalAgricultural SciencesSciences andand NaturalNatural ResourcesResources OklahomaOklahoma StateState UniversityUniversity Arthropod Pest Management in Greenhouses and Interiorscapes E-1011 Eric J. Rebek Extension Entomologist/ Ornamentals and Turfgrass Specialist Michael A. Schnelle Extension Ornamentals/ Floriculture Specialist ArthropodArthropod PestPest ManagementManagement inin GreenhousesGreenhouses andand InteriorscapesInteriorscapes Insects and their relatives cause major plant ing a hand lens. damage in commercial greenhouses and interi- Aphids feed on buds, leaves, stems, and roots orscapes. Identification of key pests and an un- by inserting their long, straw-like, piercing-suck- derstanding of appropriate control measures are ing mouthparts (stylets) and withdrawing plant essential to guard against costly crop losses. With sap. Expanding leaves from damaged buds may be tightening regulations on conventional insecti- curled or twisted and attacked leaves often display cides and increasing consumer sensitivity to their chlorotic (yellow-white) speckles where cell con- use in public spaces, growers must seek effective tents have been removed. A secondary problem pest management alternatives to conventional arises from sugary honeydew excreted by aphids. chemical control. Management strategies cen- Leaves may appear shiny and become sticky from tered around -
Shortterm Heat Stress Results in Diminution of Bacterial Symbionts
Short-term heat stress results in diminution of bacterial symbionts but has little effect on life history in adult female citrus mealybugs Article (Accepted Version) Parkinson, Jasmine F, Gobin, Bruno and Hughes, William O H (2014) Short-term heat stress results in diminution of bacterial symbionts but has little effect on life history in adult female citrus mealybugs. Entomologia Experimentalis et Applicata, 153 (1). pp. 1-9. ISSN 0013-8703 This version is available from Sussex Research Online: http://sro.sussex.ac.uk/id/eprint/60196/ This document is made available in accordance with publisher policies and may differ from the published version or from the version of record. If you wish to cite this item you are advised to consult the publisher’s version. Please see the URL above for details on accessing the published version. Copyright and reuse: Sussex Research Online is a digital repository of the research output of the University. Copyright and all moral rights to the version of the paper presented here belong to the individual author(s) and/or other copyright owners. To the extent reasonable and practicable, the material made available in SRO has been checked for eligibility before being made available. Copies of full text items generally can be reproduced, displayed or performed and given to third parties in any format or medium for personal research or study, educational, or not-for-profit purposes without prior permission or charge, provided that the authors, title and full bibliographic details are credited, a hyperlink and/or URL is given for the original metadata page and the content is not changed in any way. -
The Passionvine Mealybug, Planococcus Minor (Maskell) (Hemiptera: Pseudococcidae), and Its Natural Enemies in the Cocoa Agroecosystem in Trinidad ⇑ Antonio W
Biological Control 60 (2012) 290–296 Contents lists available at SciVerse ScienceDirect Biological Control journal homepage: www.elsevier.com/locate/ybcon The passionvine mealybug, Planococcus minor (Maskell) (Hemiptera: Pseudococcidae), and its natural enemies in the cocoa agroecosystem in Trinidad ⇑ Antonio W. Francis a, , Moses T.K. Kairo a, Amy L. Roda b, Oscar E. Liburd c, Perry Polar d a Florida A&M University, College of Engineering Sciences, Technology, and Agriculture, Center for Biological Control, Tallahassee, FL 32304, USA b USDA-APHIS-PPQ-Center for Plant Health Science and Technology, Miami, FL 33158, USA c Department of Entomology and Nematology, University of Florida, Gainesville, FL 32611, USA d Caribbean Network for Land and Urban Management, The University of the West Indies, St. Augustine, Trinidad highlights graphical abstract " Planococcus minor is found in Trinidad where little was known about the pest. " The mealybug was widely distributed on cocoa and infestation levels were low. " Cocoa field sites were surveyed for natural enemies. " We identified key natural enemies attacking the mealybug. " Their identification is a key step in the biological control process. article info abstract Article history: Planococcus minor (Maskell) is native to South Asia, but it is also present in several Neotropical locations Received 11 August 2011 including the island of Trinidad in the southern Caribbean. The mealybug poses a serious threat to unin- Accepted 2 December 2011 fested countries in this region as well as the mainland U.S. As part of an effort to gather much needed Available online 13 December 2011 information on P. minor, 33 cocoa (Theobroma cacao L.) field sites on the island were surveyed in 2006 with a view to assess the occurrence and pest status of the mealybug. -
Insect Control Update
Insect Control Update Diane Alston Utah State University Extension 2006 Pesticide Recertification Workshops Topics ◘ Pest – Japanese Beetle ◘ Insect Diagnostics – Recognizing Common Insects & Plant Injury ◘ Examples of Insect Pests ◘ Woody Ornamentals ◘ Greenhouse ◘ Turf Japanese Beetle Popillia japonica Scarab Beetle First found in U.S. in 1916 Orem, Utah: July 2006 >600 adults Mating pair of adults Trap: Sex pheromone/ Floral lure Adult feeding injury to Virginia Creeper Japanese Beetle Primarily a turf pest – Larvae or grubs feed on grass roots Adults have a broad host range – Skeletonize leaves – rose, fruit trees, shade trees, grape, etc. Injury to rose Injury to crabapple Japanese Beetle Management ◘ Eradication is extremely difficult ◘ Don’t panic – it’s unlikely to have a large impact ◘ Keep plants healthy ◘ Plant non-attractive plants (lilac, forsythia, dogwood, magnolia, American Holly) ◘ If detected in turf, control larvae with insecticides (imidacloprid, carbaryl, permethrin) ◘ Traps can provide some adult suppression (75% catch; but can attract them into an area) ◘ Contact local Utah Dept. of Agriculture and Food Office Japanese Beetle Fact Sheet on USU Extension Web Site http://extension.usu.edu/files/publications/factsheet/ENT-100-06PR-A.pdf Insect Diagnosis Insect is present Injury is present What type of injury? Friend or Foe? What life stage is present? Insect Feeding Types Borers Chewing Piercing-Sucking Gall Formers Diagnosis Scouting for Pests ◘ Look at the big picture ◘ Pattern of plant decline/injury ◘ Pest injury -
MF3001 Mealybug
i Mealybug Management in Greenhouses and Interiorscapes Mealybugs are major insect pests of greenhouse and interiorscape environments (including conservatories) where they feed on a wide range of plants and are difficult to manage (suppress) with insecticides. Host plant range depends on the particular mealybug species but includes herbaceous annuals or perennials, foliage plants, orchids, vegetables, and herbs. Specific plants include aglaonema, begonia, chrysanthemum, coleus (Solenostemon scutel- larioides), croton (Codiaeum variegatum), dracaena, false aralia (Dizygotheca elegantissima), ficus, grape ivy (Cissus rhombifolia), marigold, poinsettia (Euphorbia pulcherrima), pothos (Epipremnum aureum), and transvaal daisy (Gerbera jamesonii). A number of mealybug species may be found in green- Figure 3. Mealybug life cycle houses and interiorscapes, but the predominant species are the citrus mealybug, Planococcus citri and the longtailed (Figure 4), and do not mealybug, Pseudococcus longispinus. In addition to these have to mate to repro- two species, which feed aboveground, root mealybugs duce (this is referred (Rhizoecus spp.) are of concern because they are extremely to as parthenogen- difficult to detect and manage with available insecticides. esis). Eggs hatch into Biology and Damage crawlers that actively move around seeking Mealybugs are elliptical in shape with white, waxy protru- places to settle and feed. sions extending from the body (Figure 1). Females are Crawlers are yellow- white, wingless and 2 to 5 mm long when full-grown, Figure 4. Long-tailed mealybugs orange (Figure 5), even- (Figure 2). Males are tually turning white af- typically smaller. Most ter each successive molt. mealybug species Once settled, mealybugs reproduce asexually progress through several (lay eggs). The typi- growth stages before cal female mealybug becoming adults. -
Honeydew Collecting in Malagasy Stingless Bees (Hymenoptera: Apidae: Meliponini) and Observations on Competition with Invasive Ants
See discussions, stats, and author profiles for this publication at: https://www.researchgate.net/publication/232669144 Honeydew Collecting in Malagasy Stingless Bees (Hymenoptera: Apidae: Meliponini) and Observations on Competition with Invasive Ants Article in African Entomology · April 2011 DOI: 10.4001/003.019.0111 CITATIONS READS 6 127 3 authors, including: Hauke Koch Marlotte Jonker University of Texas at Austin University of Freiburg 33 PUBLICATIONS 891 CITATIONS 3 PUBLICATIONS 15 CITATIONS SEE PROFILE SEE PROFILE Some of the authors of this publication are also working on these related projects: TBA course View project ConFoBi - Conservation of Forest Biodiversity in Multiple-use Landscapes of Central Europe View project All content following this page was uploaded by Hauke Koch on 17 August 2014. The user has requested enhancement of the downloaded file. Honeydew collecting in Malagasy stingless bees (Hymenoptera: Apidae: Meliponini) and observations on competition with invasive ants H. Koch1*, C. Corcoran2 & M. Jonker3 1Experimental Ecology, Institute for Integrative Biology, ETH Zurich, Universitätsstrasse 16, 8092 Zurich, Switzerland 2Trinity College Dublin, Dublin 2, Ireland 3Resource Ecology Group, Wageningen University, Droevendaalsesteeg 3a, 6708PB Wageningen, Netherlands We present the first record of honeydew feeding in Malagasy stingless bees. Two species of stingless bees, Liotrigona mahafalya and L. madecassa, collected honeydew produced by mealybugs on an Albizia perrieri (Fabaceae) tree in the dry deciduous forest of Kirindy, Madagascar. Honeydew might represent an important part of the diet of Malagasy stingless bees, especially in times of scarce floral resources in the highly seasonal environment of western Madagascar. The interaction between the bees and two species of invasive ants, Monomorium destructor and Paratrechina longicornis, in competition for the honeydew resource, was studied. -
Trophobiosis Between Formicidae and Hemiptera (Sternorrhyncha and Auchenorrhyncha): an Overview
December, 2001 Neotropical Entomology 30(4) 501 FORUM Trophobiosis Between Formicidae and Hemiptera (Sternorrhyncha and Auchenorrhyncha): an Overview JACQUES H.C. DELABIE 1Lab. Mirmecologia, UPA Convênio CEPLAC/UESC, Centro de Pesquisas do Cacau, CEPLAC, C. postal 7, 45600-000, Itabuna, BA and Depto. Ciências Agrárias e Ambientais, Univ. Estadual de Santa Cruz, 45660-000, Ilhéus, BA, [email protected] Neotropical Entomology 30(4): 501-516 (2001) Trofobiose Entre Formicidae e Hemiptera (Sternorrhyncha e Auchenorrhyncha): Uma Visão Geral RESUMO – Fêz-se uma revisão sobre a relação conhecida como trofobiose e que ocorre de forma convergente entre formigas e diferentes grupos de Hemiptera Sternorrhyncha e Auchenorrhyncha (até então conhecidos como ‘Homoptera’). As principais características dos ‘Homoptera’ e dos Formicidae que favorecem as interações trofobióticas, tais como a excreção de honeydew por insetos sugadores, atendimento por formigas e necessidades fisiológicas dos dois grupos de insetos, são discutidas. Aspectos da sua evolução convergente são apresenta- dos. O sistema mais arcaico não é exatamente trofobiótico, as forrageadoras coletam o honeydew despejado ao acaso na folhagem por indivíduos ou grupos de ‘Homoptera’ não associados. As relações trofobióticas mais comuns são facultativas, no entanto, esta forma de mutualismo é extremamente diversificada e é responsável por numerosas adaptações fisiológicas, morfológicas ou comportamentais entre os ‘Homoptera’, em particular Sternorrhyncha. As trofobioses mais diferenciadas são verdadeiras simbioses onde as adaptações mais extremas são observadas do lado dos ‘Homoptera’. Ao mesmo tempo, as formigas mostram adaptações comportamentais que resultam de um longo período de coevolução. Considerando-se os inse- tos sugadores como principais pragas dos cultivos em nível mundial, as implicações das rela- ções trofobióticas são discutidas no contexto das comunidades de insetos em geral, focalizan- do os problemas que geram em Manejo Integrado de Pragas (MIP), em particular.