Quantification of RNA Polymerase I Transcriptional Attenuation at the Active VSG Expression Site in Trypanosoma Brucei
Total Page:16
File Type:pdf, Size:1020Kb
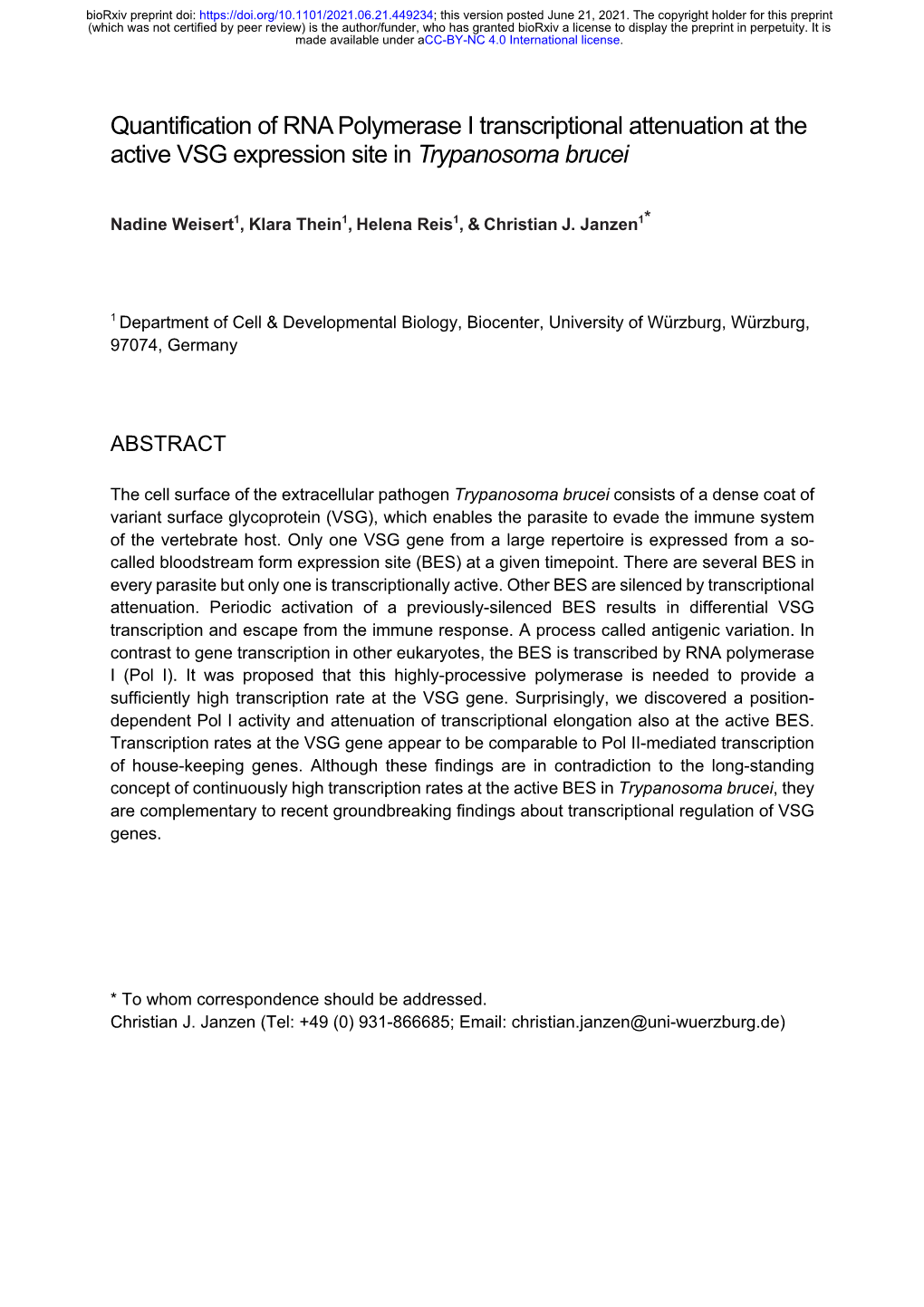
Load more
Recommended publications
-
Utility of Subtelomeric Fluorescent DNA Probes for Detection of Chromosome Anomalies in 425 Patients Syed M
article January/February 2003 ⅐ Vol. 5 ⅐ No. 1 Utility of subtelomeric fluorescent DNA probes for detection of chromosome anomalies in 425 patients Syed M. Jalal, PhD1, Aaron R. Harwood1, Gurbax S. Sekhon, PhD3, Cindy Pham Lorentz, MS1, Rhett P. Ketterling, MD1, Dusica Babovic-Vuksanovic, MD2, Reid G. Meyer1, Regina Ensenauer, MD2, Marvin H. Anderson, Jr1, and Virginia V. Michels, MD2 Purpose: A complete set of subtelomeric fluorescent DNA probes, except the acrocentric p-arms, was developed in 1996, was optimized in 1998, and is commercially available. These and other fluorescence in situ hybridization (FISH) probes have been used to detect anomalies of the subtelomere regions among groups of patients with idiopathic mental retardation (MR), developmental delay (DD), and/or nonspecific dysmorphic features (NDF), and individuals with multiple miscarriages (MM) who were karyotypically normal by standard G-banding techniques. Methods: A total of 425 patients were analyzed, of whom 372 had idiopathic MR/DD/NDF and 53 were involved in MM. An effort was made to select individuals for this study who were either normal karyotypically or who had subtle chromosomal anomalies that were inconclusive by banded chromosome analysis, although this was not always possible. Results: Anomalies involving the subtelomere regions were detected at a frequency of 6.8% in the MR/DD/NDF group. The cryptic or subtle anomalies are estimated to be about 3.4%. It was necessary to use M-FISH, chromosome, and locus specific FISH probes to clarify some of the abnormalities. No abnormalities were detected in the MM group. Deletion variants were present for 2qter, 7pter, and Xpter/Ypter subtelomeric regions ranging from Ͻ1 to 9.6%. -
Harnessing Single-Molecule Sequencing to Characterize the Fast-Evolving Drosophila Subtelomere Xander M
Harnessing single-molecule sequencing to characterize the fast-evolving Drosophila subtelomere Xander M. Gottfried, COL 2021 Mia T. Levine, College of Arts and Sciences Department of Biology Abstract ORF polymorphism is concentrated closer to telomere The telomere and subtelomere are repetitive sequences at the ends of chromosomes Use genome BLAST to find subtelomeric genes required for chromosome length preservation. In Drosophila, telomere and subtelomere are highly plastic; each of them varies in copy number and sequence both within and across species. In addition, there is evidence of functional crosstalk between telomere and subtelomere, suggesting that the two regions may co-evolve to maintain system fidelity. However, without characterizing the sequence of the subtelomere, we cannot investigate whether subtelomere evolution affects telomere function. This characterization has recently been made possible due to the advent of single-molecule sequencing, which can be used to assemble repetitive regions using long, 100 kilobase reads. Here, we begin to characterize the composition and variability of subtelomeric genes, focusing on exon duplications, intergenic distance variability, and functional open reading frame polymorphism. The Drosophila Subtelomere • Highly variable in copy number and sequence within species • Rapidly evolving Exon fragment duplications are more common closer across species to the telomere • Pervasive terminal Chromosome 2L Average # Exon Fragment Duplications Across Genomes 12 deletions • Functional Experimental Validation: crosstalk with 8 • PCR: primers to absent genes, primers to unorthodox telomere has break points, primers across gaps implications for • Cell biology: DNA FISH to gene sequences, IF to 4 genome integrity proteins RNAseq to dysfunctional ORFs Average # ofExon FragmentDuplications # Average 0 References: Anderson, J.A., Song, Y.S., and Langley, C.H. -
Epigenetic Characteristics of Human Subtelomeres Vary in Cells Utilizing the Alternative Lengthening of Telomeres (ALT) Pathway
life Article Epigenetic Characteristics of Human Subtelomeres Vary in Cells Utilizing the Alternative Lengthening of Telomeres (ALT) Pathway Shir Toubiana 1,† , Aya Tzur-Gilat 1,† and Sara Selig 1,2,* 1 Department of Genetics and Developmental Biology, Rappaport Faculty of Medicine and Research Institute, Technion, Haifa 31096, Israel; [email protected] (S.T.); [email protected] (A.T.-G.) 2 Laboratory of Molecular Medicine, Rambam Health Care Campus, Haifa 31096, Israel * Correspondence: [email protected] † Both authors contributed equally. Abstract: Most human cancers circumvent senescence by activating a telomere length maintenance mechanism, most commonly involving telomerase activation. A minority of cancers utilize the recombination-based alternative lengthening of telomeres (ALT) pathway. The exact requirements for unleashing normally repressed recombination at telomeres are yet unclear. Epigenetic modifications at telomeric regions were suggested to be pivotal for activating ALT; however, conflicting data exist regarding their exact nature and necessity. To uncover common ALT-positive epigenetic characteristics, we performed a comprehensive analysis of subtelomeric DNA methylation, histone modifications, and TERRA expression in several ALT-positive and ALT-negative cell lines. We found that subtelomeric DNA methylation does not differentiate between the ALT-positive and ALT- negative groups, and most of the analyzed subtelomeres within each group do not share common Citation: Toubiana, S.; Tzur-Gilat, A.; DNA methylation patterns. Additionally, similar TERRA levels were measured in the ALT-positive Selig, S. Epigenetic Characteristics of and ALT-negative groups, and TERRA levels varied significantly among the members of the ALT- Human Subtelomeres Vary in Cells positive group. Subtelomeric H3K4 and H3K9 trimethylation also differed significantly between Utilizing the Alternative Lengthening samples in the ALT-positive group. -
Subtelomere Organization in the Genome of the Microsporidian Encephalitozoon Cuniculi: Patterns of Repeated Sequences and Physic
Dia et al. BMC Genomics (2016) 17:34 DOI 10.1186/s12864-015-1920-7 RESEARCH ARTICLE Open Access Subtelomere organization in the genome of the microsporidian Encephalitozoon cuniculi: patterns of repeated sequences and physicochemical signatures Ndongo Dia1*, Laurence Lavie2, Ngor Faye3, Guy Méténier2, Edouard Yeramian4, Christophe Duroure5, Bhen S. Toguebaye3, Roger Frutos6, Mbayame N. Niang1, Christian P. Vivarès2, Choukri Ben Mamoun7 and Emmanuel Cornillot8,9* Abstract Background: The microsporidian Encephalitozoon cuniculi is an obligate intracellular eukaryotic pathogen with a small nuclear genome (2.9 Mbp) consisting of 11 chromosomes. Although each chromosome end is known to contain a single rDNA unit, the incomplete assembly of subtelomeric regions following sequencing of the genome identified only 3 of the 22 expected rDNA units. While chromosome end assembly remains a difficult process in most eukaryotic genomes, it is of significant importance for pathogens because these regions encode factors important for virulence and host evasion. Results: Here we report the first complete assembly of E. cuniculi chromosome ends, and describe a novel mosaic structure of segmental duplications (EXT repeats) in these regions. EXT repeats range in size between 3.5 and 23.8 kbp and contain four multigene families encoding membrane associated proteins. Twenty-one recombination sites were identified in the sub-terminal region of E. cuniculi chromosomes. Our analysis suggests that these sites contribute to the diversity of chromosome ends organization through Double Strand Break repair mechanisms. The region containing EXT repeats at chromosome extremities can be differentiated based on gene composition, GC content, recombination sites density and chromosome landscape. Conclusion: Together this study provides the complete structure of the chromosome ends of E. -
Evidence-Based Guideline: Evaluation, Diagnosis, and Management Of
Evidence-based Guideline: Evaluation, Diagnosis, and Management of Facioscapulohumeral Muscular Dystrophy Report of the Guideline Development, Dissemination, and Implementation Subcommittee of the American Academy of Neurology and the Practice Issues Review Panel of the American Association of Neuromuscular & Electrodiagnostic Medicine Rabi Tawil, MD, FAAN1; John T. Kissel, MD, FAAN2; Chad Heatwole, MD, MS-CI3; Shree Pandya, PT, DPT, MS4; Gary Gronseth, MD, FAAN5; Michael Benatar, MBChB, DPhil, FAAN6 (1) MDA Neuromuscular Disease Clinic, School of Medicine and Dentistry, University of Rochester Medical Center, Rochester, NY (2) Department of Neurology, Wexner Medical Center, Ohio State University, Columbus, OH (3) Department of Neurology, School of Medicine and Dentistry, University of Rochester Medical Center, Rochester, NY (4) Department of Neurology, School of Medicine and Dentistry, University of Rochester Medical Center, Rochester, NY (5) Department of Neurology, University of Kansas School of Medicine, Kansas City, KS (6) Department of Neurology, Miller School of Medicine, University of Miami, Miami, FL Correspondence to: American Academy of Neurology [email protected] 1 Approved by the Guideline Development, Dissemination, and Implementation Subcommittee on July 23, 2014; by the AAN Practice Committee on October 20, 2014; by the AANEM Board of Directors on [date]; and by the AANI Board of Directors on [date]. This guideline was endorsed by the FSH Society on December 18, 2014. 2 AUTHOR CONTRIBUTIONS Rabi Tawil: study concept and design, acquisition of data, analysis or interpretation of data, drafting/revising the manuscript, critical revision of the manuscript for important intellectual content, study supervision. John Kissel: acquisition of data, analysis or interpretation of data, critical revision of the manuscript for important intellectual content. -
Human Subtelomeric Duplicon Structure and Organization Comment Anthony Ambrosini*†, Sheila Paul*, Sufen Hu* and Harold Riethman*
Open Access Research2007AmbrosinietVolume al. 8, Issue 7, Article R151 Human subtelomeric duplicon structure and organization comment Anthony Ambrosini*†, Sheila Paul*, Sufen Hu* and Harold Riethman* Addresses: *The Wistar Institute, Spruce St, Philadelphia, PA 19104, USA. †Department of Molecular Biology, Princeton University, Princeton, NJ 08544, USA. Correspondence: Harold Riethman. Email: [email protected] reviews Published: 30 July 2007 Received: 29 March 2007 Revised: 25 June 2007 Genome Biology 2007, 8:R151 (doi:10.1186/gb-2007-8-7-r151) Accepted: 30 July 2007 The electronic version of this article is the complete one and can be found online at http://genomebiology.com/2007/8/7/R151 © 2007 Ambrosini et al.; licensee BioMed Central Ltd. This is an open access article distributed under the terms of the Creative Commons Attribution License (http://creativecommons.org/licenses/by/2.0), which permits unrestricted use, distribution, and reproduction in any medium, provided the original work is properly cited. Subtelomere<p>Thetelomere sequence alleles; structure a divergenceclass of duplicon within blocks subtelomeric was identified duplicon that families are subtelomere-specific.</p> varies considerably, as does the organization of duplicon blocks at sub- reports Abstract Background: Human subtelomeric segmental duplications ('subtelomeric repeats') comprise deposited research about 25% of the most distal 500 kb and 80% of the most distal 100 kb in human DNA. A systematic analysis of the duplication substructure of human subtelomeric regions was done in order to develop a detailed understanding of subtelomeric sequence organization and a nucleotide sequence-level characterization of subtelomeric duplicon families. Results: The extent of nucleotide sequence divergence within subtelomeric duplicon families varies considerably, as does the organization of duplicon blocks at subtelomere alleles. -
Analysis of the VSG Gene Silent Archive in Trypanosoma Brucei
Downloaded from genome.cshlp.org on September 30, 2021 - Published by Cold Spring Harbor Laboratory Press Letter Analysis of the VSG gene silent archive in Trypanosoma brucei reveals that mosaic gene expression is prominent in antigenic variation and is favored by archive substructure Lucio Marcello and J. David Barry1 Wellcome Centre for Molecular Parasitology, University of Glasgow, Glasgow Biomedical Research Centre, Glasgow G12 8TA, United Kingdom Trypanosoma brucei evades host acquired immunity through differential activation of its large archive of silent variant surface glycoprotein (VSG) genes, most of which are pseudogenes in subtelomeric arrays. We have analyzed 940 VSGs, representing one half to two thirds of the arrays. Sequence types A and B of the VSG N-terminal domains were confirmed, while type C was found to be a constituent of type A. Two new C-terminal domain types were found. Nearly all combinations of domain types occurred, with some bias to particular combinations. One-third of encoded N-terminal domains, but only 13% of C-terminal domains, are intact, indicating a particular need for silent VSGs to gain a functional C-terminal domain to be expressed. About 60% of VSGs are unique, the rest occurring in subfamilies of two to four close homologs (>50%–52% peptide identity). We found a subset of VSG-related genes, differing from VSGs in genomic environment and expression patterns, and predict they have distinct function. Almost all (92%) full-length array VSGs have the partially conserved flanks associated with the duplication mechanism that activates silent genes, and these sequences have also contributed to archive evolution, mediating most of the conversions of segments, containing Ն1 VSG, within and between arrays. -
Contrasting Evolutionary Genome Dynamics Between Domesticated and Wild Yeasts
ARTICLES OPEN Contrasting evolutionary genome dynamics between domesticated and wild yeasts Jia-Xing Yue1 , Jing Li1, Louise Aigrain2, Johan Hallin1 , Karl Persson3 , Karen Oliver2, Anders Bergström2, Paul Coupland2,5, Jonas Warringer3 , Marco Cosentino Lagomarsino4, Gilles Fischer4, Richard Durbin2 & Gianni Liti1 Structural rearrangements have long been recognized as an important source of genetic variation, with implications in phenotypic diversity and disease, yet their detailed evolutionary dynamics remain elusive. Here we use long-read sequencing to generate end- to-end genome assemblies for 12 strains representing major subpopulations of the partially domesticated yeast Saccharomyces cerevisiae and its wild relative Saccharomyces paradoxus. These population-level high-quality genomes with comprehensive annotation enable precise definition of chromosomal boundaries between cores and subtelomeres and a high-resolution view of evolutionary genome dynamics. In chromosomal cores, S. paradoxus shows faster accumulation of balanced rearrangements (inversions, reciprocal translocations and transpositions), whereas S. cerevisiae accumulates unbalanced rearrangements (novel insertions, deletions and duplications) more rapidly. In subtelomeres, both species show extensive interchromosomal reshuffling, with a higher tempo in S. cerevisiae. Such striking contrasts between wild and domesticated yeasts are likely to reflect the influence of human activities on structural genome evolution. Understanding how genetic variation translates into phenotypic biology and genetics. It was the first eukaryote to have its genome diversity is a central theme in biology. With the rapid advancement of sequence, population genomics and genotype–phenotype map exten- sequencing technology, genetic variation in large natural populations sively explored1,20,21. Here we applied PacBio sequencing to 12 repre- has been explored extensively for humans and several model organ- sentative strains of S. -
Integration Specificity of the Retrovirus-Like Transposable Element Ty5 of Saccharomyces Sige Zou Iowa State University
Iowa State University Capstones, Theses and Retrospective Theses and Dissertations Dissertations 1996 Integration specificity of the retrovirus-like transposable element Ty5 of Saccharomyces Sige Zou Iowa State University Follow this and additional works at: https://lib.dr.iastate.edu/rtd Part of the Genetics Commons, Microbiology Commons, and the Molecular Biology Commons Recommended Citation Zou, Sige, "Integration specificity of the retrovirus-like transposable element Ty5 of Saccharomyces " (1996). Retrospective Theses and Dissertations. 11353. https://lib.dr.iastate.edu/rtd/11353 This Dissertation is brought to you for free and open access by the Iowa State University Capstones, Theses and Dissertations at Iowa State University Digital Repository. It has been accepted for inclusion in Retrospective Theses and Dissertations by an authorized administrator of Iowa State University Digital Repository. For more information, please contact [email protected]. INFORMATION TO USERS This manuscript has been reproduced from the microfilm master. UMI fihns the text directly fiom the original or copy submitted. Thus, some theas and dissertation copies are in typewriter &ce, vtliile others may be from ai^ type of computer printer. The quality of this reproduction is dependent upon the quality of the copy submitted. Broken or indistinct print, colored or poor quality illustrations and photographs, print bleedthrough, substandard margins, and improper alignment can adversely affect rq)roduction. In the unlikdy event that the author did not send UMI a complete nuuiuscript and there are misdng pages, these will be noted. Also, if unauthorized copyright material had to be removed, a note will indicate the deletion. Oversize materials (e.g., m^s, drawings, charts) are reproduced by sectioning the original, beginning at the upper left-hand comer and continuing from left to right in equal sections with small overlaps. -
Retrotransposons That Maintain Chromosome Ends
SACKLER SPECIAL FEATURE: PERSPECTIVE Retrotransposons that maintain chromosome ends Mary-Lou Pardue1 and P. G. DeBaryshe Department of Biology, Massachusetts Institute of Technology, Cambridge, MA 02139 Edited by Joan Curcio, Wadsworth Center, New York State Department of Health, Albany, NY, and accepted by the Editorial Board June 28, 2011 (received for review January 30, 2011) Reverse transcriptases have shaped genomes in many ways. A remarkable example of this shaping is found on telomeres of the genus Drosophila, where retrotransposons have a vital role in chromosome structure. Drosophila lacks telomerase; instead, three telomere- specific retrotransposons maintain chromosome ends. Repeated transpositions to chromosome ends produce long head to tail arrays of these elements. In both form and function, these arrays are analogous to the arrays of repeats added by telomerase to chromosomes in other organisms. Distantly related Drosophila exhibit this variant mechanism of telomere maintenance, which was established before the separation of extant Drosophila species. Nevertheless, the telomere-specific elements still have the hallmarks that characterize non-long terminal repeat (non-LTR) retrotransposons; they have also acquired characteristics associated with their roles at telomeres. These telomeric retrotransposons have shaped the Drosophila genome, but they have also been shaped by the genome. Here, we discuss ways in which these three telomere-specific retrotransposons have been modified for their roles in Drosophila chromosomes. centromeres | chromosome evolution | heterochromatin | euchromatin | Y chromosome ells invest an unexpected amount HeT-A, TART, and TAHRE: A Ménage cessive transpositions produce long arrays of their resources in what might à Trois? of these elements, all oriented with their Cseem to be relatively insignificant Like other complex repeat sequences, 5′ ends toward the end of the chromo- parts of the chromosome, their telomere database entries are prone to some, and many showing some truncation telomeres. -
Chromosome Structure: DNA Nucleotide Sequence Elements of a Subset of the Minichromosomes of the Protozoan Trypanosoma Brucei
MOLECULAR AND CELLULAR BIOLOGY, Aug. 1991, p. 3823-3834 Vol. 11, No. 8 0270-7306/91/083823-12$02.00/0 Copyright C) 1991, American Society for Microbiology Chromosome Structure: DNA Nucleotide Sequence Elements of a Subset of the Minichromosomes of the Protozoan Trypanosoma brucei MICHAEL WEIDEN,1 YVONNE N. OSHEIM,2 ANN L. BEYER,2 AND LEX H. T. VAN DER PLOEG3* Department of Medicine' and Department of Genetics and Development,3 College ofPhysicians and Surgeons, Columbia University, 701 West 168 Street, New York, New York 10032, and Department of Microbiology, University of Virginia, Charlottesville,. Virginia 229082 Received 18 December 1990/Accepted 30 April 1991 The genome of the protozoan Trypanosoma brucei contains a set of about 100 minichromosomes of about 50 to 150 kb in size. The small size of these chromosomes, their involvement in antigenic variation, and their mitotic stability make them ideal candidates for a structural analysis of protozoan chromosomes and their telomeres. We show that a subset of the minichromosomes is composed predominantly of simple-sequence DNA, with over 90% of the length of the minichromosome consisting of a tandem array of 177-bp repeats, indicating that these molecules have limited protein-coding capacity. Proceeding from the tip of the telomere to a chromosome internal position, a subset of the minichromosomes contained the GGGTTA telomere repeat, a 29-bp telomere-derived repeat, a region containing 74-bp G+C-rich direct repeats separated by approxi- mately 155 bp of A+T-rich DNA that has a bent character, and 50 to 150 kb of the 177-bp repeat. -
Subtelomeric CTCF and Cohesin Binding Site Organization Using Improved Subtelomere Assemblies and a Novel Annotation Pipeline
Downloaded from genome.cshlp.org on September 27, 2021 - Published by Cold Spring Harbor Laboratory Press Resource Subtelomeric CTCF and cohesin binding site organization using improved subtelomere assemblies and a novel annotation pipeline Nicholas Stong,1,2 Zhong Deng,2 Ravi Gupta,2 Sufen Hu,2 Shiela Paul,2 Amber K. Weiner,2 Evan E. Eichler,3 Tina Graves,4 Catrina C. Fronick,4 Laura Courtney,4 Richard K. Wilson,4 Paul M. Lieberman,2 Ramana V. Davuluri,2 and Harold Riethman2,5 1Graduate Group in Genomics and Computational Biology, School of Medicine, University of Pennsylvania, Philadelphia, Pennsylvania 19104, USA; 2The Wistar Institute, Philadelphia, Pennsylvania 19104, USA; 3Department of Genome Sciences, University of Washington, Seattle, Washington 98195, USA; 4The Genome Institute, Washington University School of Medicine, St. Louis, Missouri 63108, USA Mapping genome-wide data to human subtelomeres has been problematic due to the incomplete assembly and challenges of low-copy repetitive DNA elements. Here, we provide updated human subtelomere sequence assemblies that were extended by filling telomere-adjacent gaps using clone-based resources. A bioinformatic pipeline incorporating multiread mapping for annotation of the updated assemblies using short-read data sets was developed and implemented. Annotation of subtelomeric sequence features as well as mapping of CTCF and cohesin binding sites using ChIP-seq data sets from multiple human cell types confirmed that CTCF and cohesin bind within 3 kb of the start of terminal repeat tracts at many, but not all, subtelomeres. CTCF and cohesin co-occupancy were also enriched near internal telomere-like sequence (ITS) islands and the nonterminal boundaries of subtelomere repeat elements (SREs) in transformed lymphoblastoid cell lines (LCLs) and human embryonic stem cell (ES) lines, but were not significantly enriched in the primary fibroblast IMR90 cell line.