Ground Vibrations Produced by Surface and Near-Surface Explosions
Total Page:16
File Type:pdf, Size:1020Kb
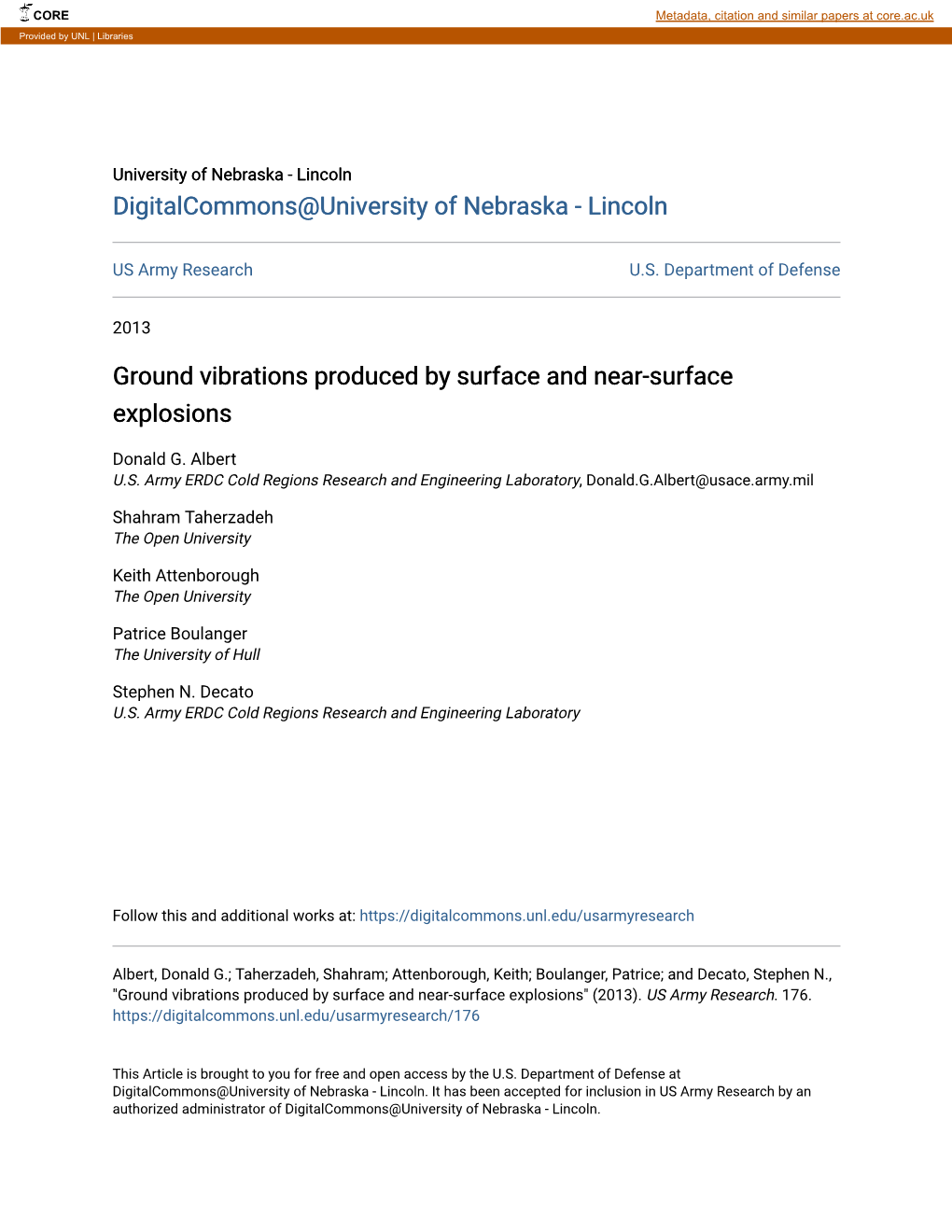
Load more
Recommended publications
-
Sources of Vibrations and Their Impact on the Environnement Abdul Karim Jamal Eddine
Sources of vibrations and their impact on the environnement Abdul Karim Jamal Eddine To cite this version: Abdul Karim Jamal Eddine. Sources of vibrations and their impact on the environnement. Géotech- nique. Université Paris-Est, 2017. English. NNT : 2017PESC1111. tel-01744297 HAL Id: tel-01744297 https://tel.archives-ouvertes.fr/tel-01744297 Submitted on 21 May 2018 HAL is a multi-disciplinary open access L’archive ouverte pluridisciplinaire HAL, est archive for the deposit and dissemination of sci- destinée au dépôt et à la diffusion de documents entific research documents, whether they are pub- scientifiques de niveau recherche, publiés ou non, lished or not. The documents may come from émanant des établissements d’enseignement et de teaching and research institutions in France or recherche français ou étrangers, des laboratoires abroad, or from public or private research centers. publics ou privés. UNIVERSITE PARIS-EST Ecole Doctorale Sciences, Ingénierie et Environnement Thèse de doctorat Présentée pour l’obtention du grade de Docteur de l’Université Paris-Est Spécialité : Génie Civil Par : Abdul Karim Jamal Eddine Sujet : Sources vibratoires et effets sur l'environnement Thèse soutenue le 25 Septembre 2017 devant le jury composé de : M. Etienne Bertrand CEREMA Rapporteur Mme Gaëlle Lefeuve-Mesgouez Université d'Avignon Rapporteure M. Emmanuel Chaljub ISTerre Examinateur M. Cyrille Fauchard CEREMA Président du jury M. Jean-François Semblat IFSTTAR Directeur de thèse M. Luca Lenti IFSTTAR Co-Directeur de thèse 1 2 3 Acknowledgments First and foremost I thank the Lord Almighty who inspired patience to finish this work and for his continuous guidance in my life. -
Ground Vibrations and Tilts
IV/336 GROUND VIBRATIONS AND TILTS Hideo Hanada National Astronomical Observatory, Mizusawa, Iwate, JAPAN 1. INTRODUCTION We cannot avoid effects of ground vibrations or tilts so far as we make some kind of precise experiments on the earth. The attraction of the Sun and the Moon periodically change positions, tilts and strains of the ground with various modes (Earth tides), ocean waves cause ground vibrations (microseisms) with periods ranging from about 2 to 10 seconds according to geology under ground, and human activities such as traffic also cause ground vibrations (cultural noises) with periods from about 0.05 to 0.2 seconds. Winds, water flows, earthquakes and volcanoes also induce vibrations although they may be very weak or local. Vibrations observed on the Earth have various frequencies ranging from 10m8 to lo2 Hz. From the point of view of spatial pattern, ground vibrations and tilts have various characteristic lengths or wavelengths being related to their periods. 2. EARTH TIDES The solid Earth always receives attraction from the Sun, the Moon and the other planets and change its shape due to them. This is called Earth tides. Since the celestial bodies are moving regularly following a certain law, variation of the potential generated by the attraction is perfectly described by a trigonometrical function with many different amplitudes, frequencies and phases.The Earth tides cause ground vibrations and tilts although their frequencies are very low. With spherical coordinates in which r be radius, 0 co-latitude, X geocentric longitude and O the center, the attractive force acting at O and P(r, 8, X) due to a celestial body B of mass M at Q( T’, 0’, X’) is expressed as where l is the distance between P and Q, cr the angle between OQ and OP, p the angle between PQ and PO, and the subscript r means the OP direction (see Figure 1). -
Ground Vibrations Near Explosions.*
GROUND VIBRATIONS NEAR EXPLOSIONS.* By BENJAMIN F. HOWELL, JR. INTRODUCTION ONE WOCLD expect that, since seismic waves from explosions are the basis of a whole industry (seismic surveying), their nature would have been throughly investigated and described. However, the exploration geophysicist is not pri- marily interested in the nature of the seismic pulses, but in their velocities and the paths they travel to his recording instruments. It is common practice in studying seismic exploration records to assume that only compressional pulses are clearly recorded, though occasionally the presence of some transverse wave energy is postulated to explain otherwise incomprehensible observations. Other types of wave motion are treated as part of the background noise, and wherever possible are excluded from the recorded spectrum by the use of ap- propriate filters in the amplifiers. However, proper interpretation of the records obtained requires some knowledge of the detailed character of the ground motion; and, therefore, any information on the nature of the seismic energy which is generated by an explosion is certain to have some value. The series of experiments described here was undertaken with the intention of increasing the knowledge of the basic seismic forms to be expected in the record of an explosion. PREVIOUS EXPERIMENTAL WORK Most of the work done in the past has been on large explosions recorded at near-by permanent seismic observatories. These investigations treat primarily data recorded many kilometers from the source of the energy. Because of the large distances, the shapes of the pulses are much altered from what they were near their source, and are not well suited to studies of their detailed nature. -
Ground Vibrations Due to Pile and Sheet Pile Driving – Influencing Factors, Predictions and Measurements
Ground vibrations due to pile and sheet pile driving – influencing factors, predictions and measurements Fanny Deckner Licentiate thesis Division of Soil and Rock Mechanics Department of Civil and Architectural Engineering School of Architecture and the Built Environment KTH, Royal Institute of Technology Stockholm 2013 TRITA‐JOB LIC 2019 ISSN 1650‐951X ISBN 978‐91‐7501‐660‐3 ©Fanny Deckner 2013 PREFACE The work presented in this thesis has been carried out between September 2009 and March 2013 at NCC Engineering and the Division of Soil and Rock Mechanics, Department of Civil and Architectural Engineering at the Royal Institute of Technology. The work was supervised by Professor Staffan Hintze with assistance from Dr Kenneth Viking. I would like to express my gratitude to the Development Fund of the Swedish Construction Industry, NCC Construction Sweden and the Royal Institute of Technology for the financial support given to this research project. I would like to gratefully acknowledge the participants in my reference group (Johan Blumfalk, Hercules; Olle Båtelsson, Trafikverket; Håkan Eriksson, GeoMind; Ulf Håkansson, Skanska/KTH, Jörgen Johansson, NGI and Nils Rydén, PEAB/LTH/KTH) for valuable comments and reflections during the process. The warmest of acknowledgements I would like to direct to my supervisors Professor Staffan Hintze and Dr Kenneth Viking. Without your support and encouragement this project would not have been possible. Furthermore, I would like to thank my wonderful colleagues at NCC Engineering for making every work day a joy. Finally, I would like to thank my beloved Joel for his great support and understanding, my wonderful son Henry for being such a happy child, the yet unborn child for letting me finish this thesis before entering the world, and the rest of my family for making this work possible.