Verde River Basin Water-Resources Primer
Total Page:16
File Type:pdf, Size:1020Kb
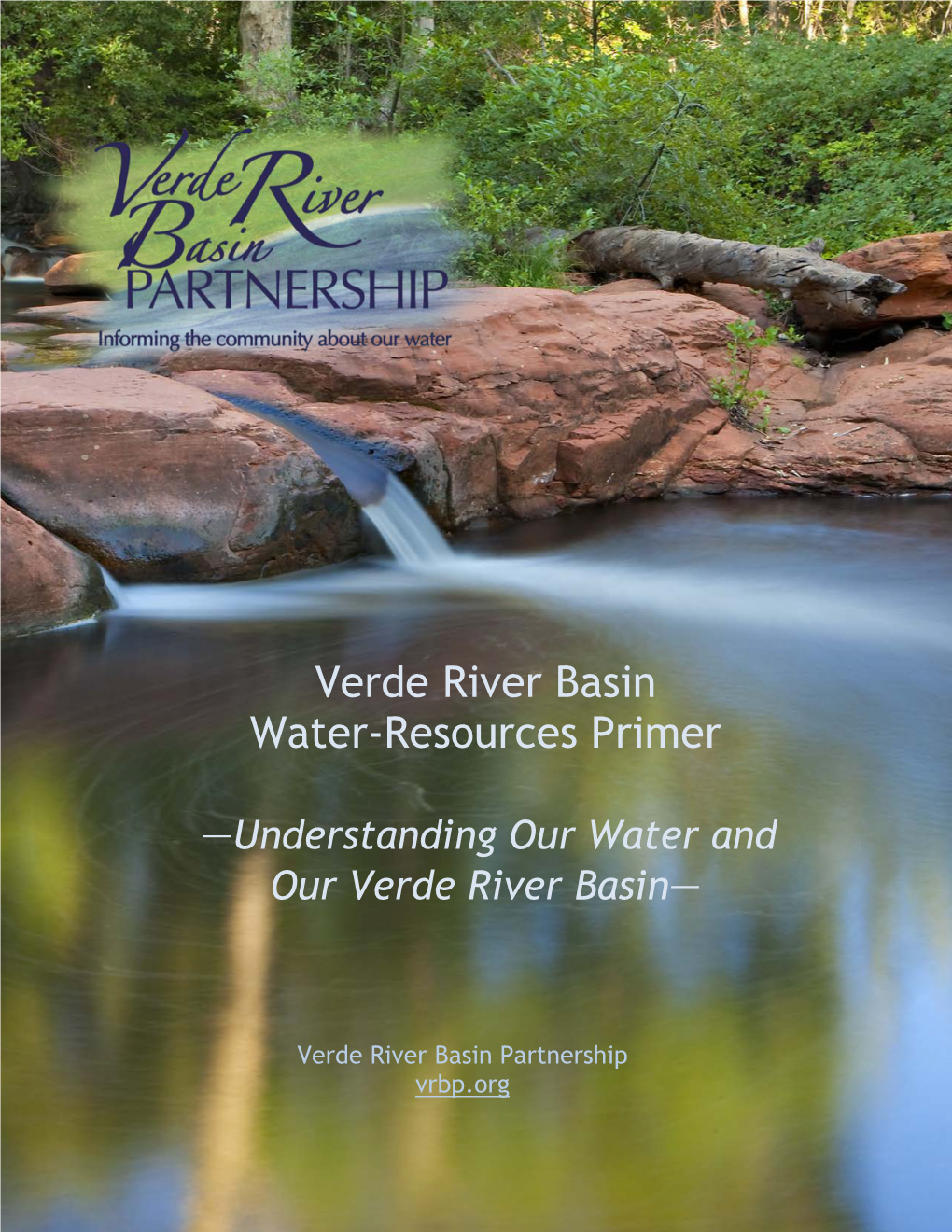
Load more
Recommended publications
-
Arizona TIM PALMER FLICKR
Arizona TIM PALMER FLICKR Colorado River at Mile 50. Cover: Salt River. Letter from the President ivers are the great treasury of noted scientists and other experts reviewed the survey design, and biological diversity in the western state-specific experts reviewed the results for each state. RUnited States. As evidence mounts The result is a state-by-state list of more than 250 of the West’s that climate is changing even faster than we outstanding streams, some protected, some still vulnerable. The feared, it becomes essential that we create Great Rivers of the West is a new type of inventory to serve the sanctuaries on our best, most natural rivers modern needs of river conservation—a list that Western Rivers that will harbor viable populations of at-risk Conservancy can use to strategically inform its work. species—not only charismatic species like salmon, but a broad range of aquatic and This is one of 11 state chapters in the report. Also available are a terrestrial species. summary of the entire report, as well as the full report text. That is what we do at Western Rivers Conservancy. We buy land With the right tools in hand, Western Rivers Conservancy is to create sanctuaries along the most outstanding rivers in the West seizing once-in-a-lifetime opportunities to acquire and protect – places where fish, wildlife and people can flourish. precious streamside lands on some of America’s finest rivers. With a talented team in place, combining more than 150 years This is a time when investment in conservation can yield huge of land acquisition experience and offices in Oregon, Colorado, dividends for the future. -
CENTRAL ARIZONA SALINITY STUDY --- PHASE I Technical Appendix C HYDROLOGIC REPORT on the PHOENIX
CENTRAL ARIZONA SALINITY STUDY --- PHASE I Technical Appendix C HYDROLOGIC REPORT ON THE PHOENIX AMA Prepared for: United States Department of Interior Bureau of Reclamation Prepared by: Brown and Caldwell 201 East Washington Street, Suite 500 Phoenix, Arizona 85004 Brown and Caldwell Project No. 23481.001 C-1 TABLE OF CONTENTS PAGE TABLE OF CONTENTS ................................................................................................................ 2 LIST OF TABLES .......................................................................................................................... 3 LIST OF FIGURES ........................................................................................................................ 3 1.0 INTRODUCTION .............................................................................................................. 4 2.0 PHYSICAL SETTING ....................................................................................................... 5 3.0 GENERALIZED GEOLOGY ............................................................................................ 6 3.1 BEDROCK GEOLOGY ......................................................................................... 6 3.2 BASIN GEOLOGY ................................................................................................ 6 4.0 HYDROGEOLOGIC CONDITIONS ................................................................................ 9 4.1 GROUNDWATER OCCURRENCE .................................................................... -
Area Land Use Plan
DETAIL VIEW #1 RIM TRAIL ESTATES DETAIL VIEW #2 GIRL SCOUT CAMP 260 KOHL'S RANCH VERDE GLEN FR 199 TONTO CREEK 5 THOMPSON THOMPSON DRAW I E. VERDE RIVER DRAW II BOY SCOUT CAMP FR 64 FR 64 WHISPERING PINES PINE MEADOWS BEAR FLATS FR 199 DETAIL VIEW #3 FLOWING SPRINGS DETAIL VIEW #4 DETAIL VIEW #5 DIAMOND POINT FOREST HOMES & 87 FR 29 COLLINS RANCH E. VERDE RIVER COCONINO COUNTY EAST VERDE PARK FR 64 260 FR 64 TONTO VILLAGE GILA COUNTYLION SPRINGS DETAIL VIEW #6 DETAIL VIEW #7 DETAIL VIEW #8 FR 200 FR FR 291 PONDEROSA SPRINGS CHRISTOPHER CREEK 260 HAIGLER CREEK HAIGLER CREEK (HIGHWAY 260 REALIGNMENT) COLCORD MOUNTAIN HOMESITES HUNTER CREEK FR 200 DETAIL VIEW #9 DETAIL VIEW #10 DETAIL VIEW #11 ROOSEVELT LAKE ESTATES 87 FR 184 188 OXBOW ESTATES SPRING CREEK 188 JAKES CORNER KEY MAP: LEGEND Residential - 3.5 to 5 du/ac Residential - 5 to 10 du/ac Regional Highways and Significant Roadways NORTHWEST NORTHEAST Major Rivers or Streams Residential - 10+ du/ac Gila County Boundary Neighborhood Commercial Community Commercial WEST EAST Federal/Incorporated Area Lands CENTRAL CENTRAL Light Industrial LAND USE CLASSIFICATIONS Heavy Industrial SOUTH Residential - 0 to 0.1 du/ac Public Facilities AREA LAND USE PLAN Residential - 0.1 to 0.4 du/ac DETAILED VIEWS Multi-Functional Corridor FIGURE 2.F Residential - 0.4 to 1.0 du/ac Mixed Use Residential - 1 to 2 du/ac Resource Conservation 0' NOVEMBER, 2003 3 Mi Residential - 2 to 3.5 du/ac GILA COUNTY COMPREHENSIVE PLAN - 2012 Potential Resort/Lodging Use 1 1/2 Mi GILA COUNTY, ARIZONA DETAIL VIEW #1 RIM TRAIL ESTATES DETAIL VIEW #2 GIRL SCOUT CAMP 260 KOHL'S RANCH VERDE GLEN FR 199 TONTO CREEK 5 THOMPSON THOMPSON DRAW I E. -
Forest Insect and Disease Conditions in the Southwestern Region, 2008
United States Department of Forest Insect and Agriculture Forest Disease Conditions in Service Southwestern the Southwestern Region Forestry and Forest Health Region, 2008 July 2009 PR-R3-16-5 The U.S. Department of Agriculture (USDA) prohibits discrimination in all its programs and activities on the basis of race, color, national origin, age, disability, and where applicable, sex, marital status, parental status, religion, sexual orientation, genetic information, political beliefs, reprisal, or because all or part of an individual’s income is derived from any public assistance program. (Not all prohibited bases apply to all programs.) Persons with disabilities who require alternative means for communication of program information (Braille, large print, audiotape, etc.) should contact USDA's TARGET Center at (202) 720- 2600 (voice and TTY). To file a complaint of discrimination, write to USDA, Director, Office of Civil Rights, 1400 Independence Avenue, SW, Washington, DC 20250-9410 or call (800) 795-3272 (voice) or (202) 720-6382 (TTY). USDA is an equal opportunity provider and employer. Cover photo: Pandora moth caterpillar collected on the North Kaibab Ranger District, Kaibab National Forest. Forest Insect and Disease Conditions in the Southwestern Region, 2008 Southwestern Region Forestry and Forest Health Regional Office Salomon Ramirez, Director Allen White, Pesticide Specialist Forest Health Zones Offices Arizona Zone John Anhold, Zone Leader Mary Lou Fairweather, Pathologist Roberta Fitzgibbon, Entomologist Joel McMillin, Entomologist -
Index 1 INDEX
Index 1 INDEX A Blue Spring 76, 106, 110, 115 Bluff Spring Trail 184 Adeii Eechii Cliffs 124 Blythe 198 Agate House 140 Blythe Intaglios 199 Agathla Peak 256 Bonita Canyon Drive 221 Agua Fria Nat'l Monument 175 Booger Canyon 194 Ajo 203 Boundary Butte 299 Ajo Mountain Loop 204 Box Canyon 132 Alamo Canyon 205 Box (The) 51 Alamo Lake SP 201 Boyce-Thompson Arboretum 190 Alstrom Point 266, 302 Boynton Canyon 149, 161 Anasazi Bridge 73 Boy Scout Canyon 197 Anasazi Canyon 302 Bright Angel Canyon 25, 51 Anderson Dam 216 Bright Angel Point 15, 25 Angels Window 27 Bright Angel Trail 42, 46, 49, 61, 80, 90 Antelope Canyon 280, 297 Brins Mesa 160 Antelope House 231 Brins Mesa Trail 161 Antelope Point Marina 294, 297 Broken Arrow Trail 155 Apache Junction 184 Buck Farm Canyon 73 Apache Lake 187 Buck Farm Overlook 34, 73, 103 Apache-Sitgreaves Nat'l Forest 167 Buckskin Gulch Confluence 275 Apache Trail 187, 188 Buenos Aires Nat'l Wildlife Refuge 226 Aravaipa Canyon 192 Bulldog Cliffs 186 Aravaipa East trailhead 193 Bullfrog Marina 302 Arch Rock 366 Bull Pen 170 Arizona Canyon Hot Springs 197 Bush Head Canyon 278 Arizona-Sonora Desert Museum 216 Arizona Trail 167 C Artist's Point 250 Aspen Forest Overlook 257 Cabeza Prieta 206 Atlatl Rock 366 Cactus Forest Drive 218 Call of the Canyon 158 B Calloway Trail 171, 203 Cameron Visitor Center 114 Baboquivari Peak 226 Camp Verde 170 Baby Bell Rock 157 Canada Goose Drive 198 Baby Rocks 256 Canyon del Muerto 231 Badger Creek 72 Canyon X 290 Bajada Loop Drive 216 Cape Final 28 Bar-10-Ranch 19 Cape Royal 27 Barrio -
Southern Sinagua Sites Tour: Montezuma Castle, Montezuma
Information as of Old Pueblo Archaeology Center Presents: March 4, 2021 99 a.m.-5:30a.m.-5:30 p.m.p.m. SouthernSouthern SinaguaSinagua SitesSites Tour:Tour: MayMay 8,8, 20212021 MontezumaMontezuma Castle,Castle, SaturdaySaturday MontezumaMontezuma Well,Well, andand TuzigootTuzigoot $30 donation ($24 for members of Old Pueblo Archaeology Center or Friends of Pueblo Grande Museum) Donations are due 10 days after reservation request or by 5 p.m. Wednesday May 8, whichever is earlier. SEE NEXT PAGES FOR DETAILS. National Park Service photographs: Upper, Tuzigoot Pueblo near Clarkdale, Arizona Middle and lower, Montezuma Well and Montezuma Castle cliff dwelling, Camp Verde, Arizona 9 a.m. to 5:30 p.m. Saturday May 8: Southern Sinagua Sites Tour – Montezuma Castle, Montezuma Well, and Tuzigoot meets at Montezuma Castle National Monument, 2800 Montezuma Castle Rd., Camp Verde, Arizona What is Sinagua? Named with the Spanish term sin agua (‘without water’), people of the Sinagua culture inhabited Arizona’s Middle Verde Valley and Flagstaff areas from about 6001400 CE Verde Valley cliff houses below the rim of Montezuma Well and grew corn, beans, and squash in scattered lo- cations. Their architecture included masonry-lined pithouses, surface pueblos, and cliff dwellings. Their pottery included some black-on-white ceramic vessels much like those produced elsewhere by the An- cestral Pueblo people but was mostly plain brown, and made using the paddle-and-anvil technique. Was Sinagua a separate culture from the sur- rounding Ancestral Pueblo, Mogollon, Hohokam, and Patayan ones? Was Sinagua a branch of one of those other cultures? Or was it a complex blending or borrowing of attributes from all of the surrounding cultures? Whatever the case might have been, today’s Hopi Indians consider the Sinagua to be ancestral to the Hopi. -
PRELIMINARY REPORT of INVESTIGATIONS of SPRINGS in the MOGOLLON RIM REGION, ARIZONA By
United States Department of the Interior Geological Survey PRELIMINARY REPORT OF INVESTIGATIONS OF SPRINGS IN THE MOGOLLON RIM REGION, ARIZONA By J. H. Feth With sections on: Base flow of streams By N. D. White and Quality of water By J. D. Hem Open-file report. Not reviewed for conformance with editorial standards of the Geological Survey. Tucson, Arizona June 1954 CONTENTS Page Abstract ................................................... 1 Introduction................................................. 3 Purpose and scope of investigation.......................... 3 Location and extent of area ................................ 4 Previous investigations.................................... 5 Personnel and acknowledgments ............................ 5 Geography .................................................. 6 Land forms and physiographic history ...................... 6 Drainage ................................................ 6 Climate ................................................. 6 Development and industry.................................. 8 Minerals"................................................. 9 Water ................................................... 9 Geology .................................................... 10 Stratigraphy ............................................. 10 Rocks of pre-Mesozoic age ............................. 10 Upper Cretaceous sedimentary rocks .................... 10 Tertiary and Quaternary sedimentary rocks .............. 11 Lake beds .......................................... 11 San Carlos basin -
Historical Geomorphology of the Verde River
Historical Geomorphology of the Verde River by Philip A. Pearthree Arizona Geological Survey Open-File Report 96-13 June, 1996 Arizona Geological Survey 416 W. Congress, Suite #100, Tucson, Arizona 85701 Research conducted in cooperation with CH2MHILL as part of the basic data collection for assessment of river navigability in Arizona at the time of Statehood (1912 J. Funding was provided by the Arizona State Land Department This report is preliminary and has not been edited or reviewed for conformity with Arizona Geological Survey standards Introduction The Verde River drainage is a major river system that heads in Big Chino Valley in north central Arizona, flows generally southeast through the rugged terrain of central Arizona, and empties into the Salt River east of the Phoenix metropolitan area. It is unusual in Arizona because much the main channel of the Verde River is perennial for much its length. Data summarized in this report were gathered to aid in the assessment of the navigability of the Verde River in February, 1912, when Arizona became a State. These investigations were conducted in cooperation with CH2MHill and were funded by the Arizona State Land Department. The purposes of this report are to (1) outline the geologic and geomorphic framework of the Verde River, (2) to describe the physical character of the channel of the Verde River, and (3) to evaluate how channel morphology and position have changed in the past century. Physiography, Geology, and General Geomorphology of the River The Verde River heads in and flows through the rugged highlands and valleys of central Arizona (Figure 1). -
June 2021 Arizona Office of Tourism Monthly State Parks Visitation Report
June 2021 Arizona Office of Tourism Monthly State Parks Visitation Report Arizona State Park Visitation June June 2021 2020 YTD State Park % Chg 2021 2020 YTD YTD % Chg Alamo Lake SP 1,237 1,924 -35.7% 48,720 46,871 3.9% Buckskin Mountain SP 11,092 9,292 19.4% 49,439 45,627 8.4% Catalina SP 6,598 2,420 172.6% 154,763 156,234 -0.9% Cattail Cove SP 10,138 14,908 -32.0% 52,221 67,256 -22.4% Colorado River SHP 167 57 193.0% 2,589 6,220 -58.4% Dead Horse Ranch SP 17,142 18,748 -8.6% 126,799 120,044 5.6% Fool Hollow Lake RA 16,380 25,104 -34.8% 55,360 63,341 -12.6% Fort Verde SHP 706 521 35.5% 4,672 2,654 76.0% Granite Mountain Hotshots MSP 1,076 1,380 -22.0% 11,778 14,874 -20.8% Homolovi SP 4,032 1,982 103.4% 22,007 11,470 91.9% Jerome SHP 4,099 1,991 105.9% 21,789 14,109 54.4% Kartchner Caverns SP 7,776 2,045 280.2% 44,020 57,663 -23.7% Lake Havasu SP 66,040 74,493 -11.3% 241,845 337,920 -28.4% Lost Dutchman SP 4,198 3,651 15.0% 122,844 132,399 -7.2% Lyman Lake SP 11,169 10,625 5.1% 30,755 38,074 -19.2% McFarland SHP 166 0 1,213 2,942 -58.8% Oracle SP 270 486 -44.4% 6,994 9,191 -23.9% Patagonia Lake SP 25,058 30,706 -18.4% 118,311 126,873 -6.7% Picacho Peak SP 1,893 1,953 -3.1% 64,084 68,800 -6.9% Red Rock SP 12,963 6,118 111.9% 56,356 33,228 69.6% Riordan Mansion SHP 777 0 2,188 2,478 -11.7% River Island SP 2,623 3,004 -12.7% 18,089 19,411 -6.8% Roper Lake SP 8,049 12,394 -35.1% 52,924 49,738 6.4% Slide Rock SP 54,922 33,491 64.0% 231,551 110,781 109.0% Tombstone Courthouse SHP 2,875 1,465 96.2% 18,261 16,394 11.4% Tonto Natural Bridge SP 8,146 7,161 13.8% 54,950 36,557 50.3% Tubac Presidio SHP 261 117 123.1% 4,240 2,923 45.1% Yuma Territorial Prison SHP 2,206 1,061 107.9% 28,672 26,505 8.2% Total All Parks 282,059 267,097 5.6% 1,647,434 1,620,577 1.7% Note: Dankworth Pond SP data is included in Roper Lake SP, Sonoita Creek SNA is included in Patagonia Lake SP and Verde River Greenway SNA is included in Dead Horse Ranch SP. -
Songbird Ecology in Southwestern Ponderosa
This file was created by scanning the printed publication. Errors identified by the software have been corrected; however, some errors may remain. Chapter 1 Ecology of Southwestern Ponderosa Pine Forests William H. Moir, Brian Geils, Mary Ann Benoit, and Dan Scurlock describes natural and human induced changes in the com- What Is Ponderosa Pine Forest position and structure of these forests. and Why Is It Important? Forests dominated by ponderosa pine (Pinus ponderosa Paleoecology var. scopulorurn) are a major forest type of western North America (figure 1; Steele 1988; Daubenmire 1978; Oliver and Ryker 1990). In this publication, a ponderosa pine The oldest remains of ponderosa pine in the Western forest has an overstory, regardless of successional stage, United States are 600,000 year old fossils found in west dominated by ponderosa pine. This definition corresponds central Nevada. Examination of pack rat middens in New to the interior ponderosa pine cover type of the Society of Mexico and Texas, shows that ponderosa pine was absent American Foresters (Eyre1980). At lower elevations in the during the Wisconsin period (about 10,400 to 43,000 years mountainous West, ponderosa pine forests are generally ago), although pinyon-juniper woodlands and mixed co- bordered by grasslands, pinyon-juniper woodlands, or nifer forests were extensive (Betancourt 1990). From the chaparral (shrublands). The ecotone may be wide or nar- late Pleistocene epoch (24,000 years ago) to the end of the row, and a ponderosa pine forest is recognized when the last ice age (about 10,400 years ago), the vegetation of the overstory contains at least 5 percent ponderosa pine (USFS Colorado Plateau moved southward or northward with 1986). -
A Conceptual Hydrogeologic Model for Fossil Springs, Western
A CONCEPTUAL HYDROGEOLOGIC MODEL FOR FOSSIL SPRINGS, WESTERN MOGOLLON RIM, ARIZONA: IMPLICATIONS FOR REGIONAL SPRINGS PROCESSES By L. Megan Green A Thesis Submitted in Partial Fulfillment of the Requirements for the Degree of Master of Science in Geology Northern Arizona University May 2008 Approved: _________________________________ Abraham E. Springer, Ph.D., Chair _________________________________ Roderic A. Parnell, Jr., Ph.D. _________________________________ Paul J. Umhoefer, Ph.D. ABSTRACT A CONCEPTUAL HYDROGEOLOGIC MODEL FOR FOSSIL SPRINGS, WEST MOGOLLON MESA, ARIZONA: IMPLICATIONS FOR REGIONAL SPRINGS PROCESSES L. Megan Green Fossil Springs is the largest spring system discharging along the western Mogollon Rim in central Arizona and is a rare and important resource to the region. The purpose of this study was to gain a better understanding of the source of groundwater discharging at Fossil Springs. This was accomplished by (1) constructing a 3-D digital hydrogeologic framework model from available data to depict the subsurface geology of the western Mogollon Rim region and (2) by compiling and interpreting regional structural and geophysical data for Arizona’s central Transition Zone. EarthVision, a 3-D GIS modeling software, was used to construct the framework model. Two end-member models were created; the first was a simple interpolation of the data and the second was a result of geologic interpretations. The second model shows a monocline trending along the Diamond Rim fault. Both models show Fossil Springs discharging at the intersection of the Diamond Rim fault and Fossil Springs fault, at the contact between the Redwall Limestone and Naco Formation. The second objective of this study was a compilation of regional data for Arizona’s central Transition Zone. -
Fossil Creek Hydrology & Travertine Geomorphology
Fossil Creek Hydrology & Travertine Geomorphology FERC Project No. 2069-003 Arizona Childs and Irving Hydropower License Prepared For: Arizona Public Service Company Phoenix, Arizona Prepared By: W.L. Bouchard & Associates, Inc. Phoenix, Arizona June 25, 1998 Fossil Creek Hydrology & Travertine Geomorphology Abstract Fossil Creek is a tributary of the Verde River in Gila and Yavapai Counties in Arizona. Stream flow is composed of a 43 cubic foot per second (cfs) base flow from Fossil Springs (a perennial source of spring flow to the creek) and runoff from precipitation which frequently occurs as destructive flash floods. These sources form 77% and 23% of the flow respectively. Fossil Springs water is supersaturated with calcium carbonate and carbon dioxide and thus tends to precipitate travertine in the upper 4 mile reach of this 14 mile stream. Travertine deposition effects stream morphology. Fossil Creek is a "flashy" stream that frequently conveys large volumes of water very quickly. Significant floods that overflow the low flow channel banks and transport significant quantities of sediment and debris Occur about every other year. These frequent flood flows tend to alter travertine deposition. Periodically, Fossil Creek is subject to very large destructive floods that significantly alter stream morphology. Arizona Public Service Company (APS) owns and operates the Childs & Irving Hydroelectric plants on Fossil Creek. The plants withdraw the 43 cfs base flow about 0.2 miles below the springs for hydropower generation. In 1992, APS proposed to the Federal Energy Regulatory Commission (FERC) to increase minimum flow releases to 10 cfs in the Irving reach and 5 cfs in the Childs reach as part of their application for relicensing.