Research.Pdf (4.530Mb)
Total Page:16
File Type:pdf, Size:1020Kb
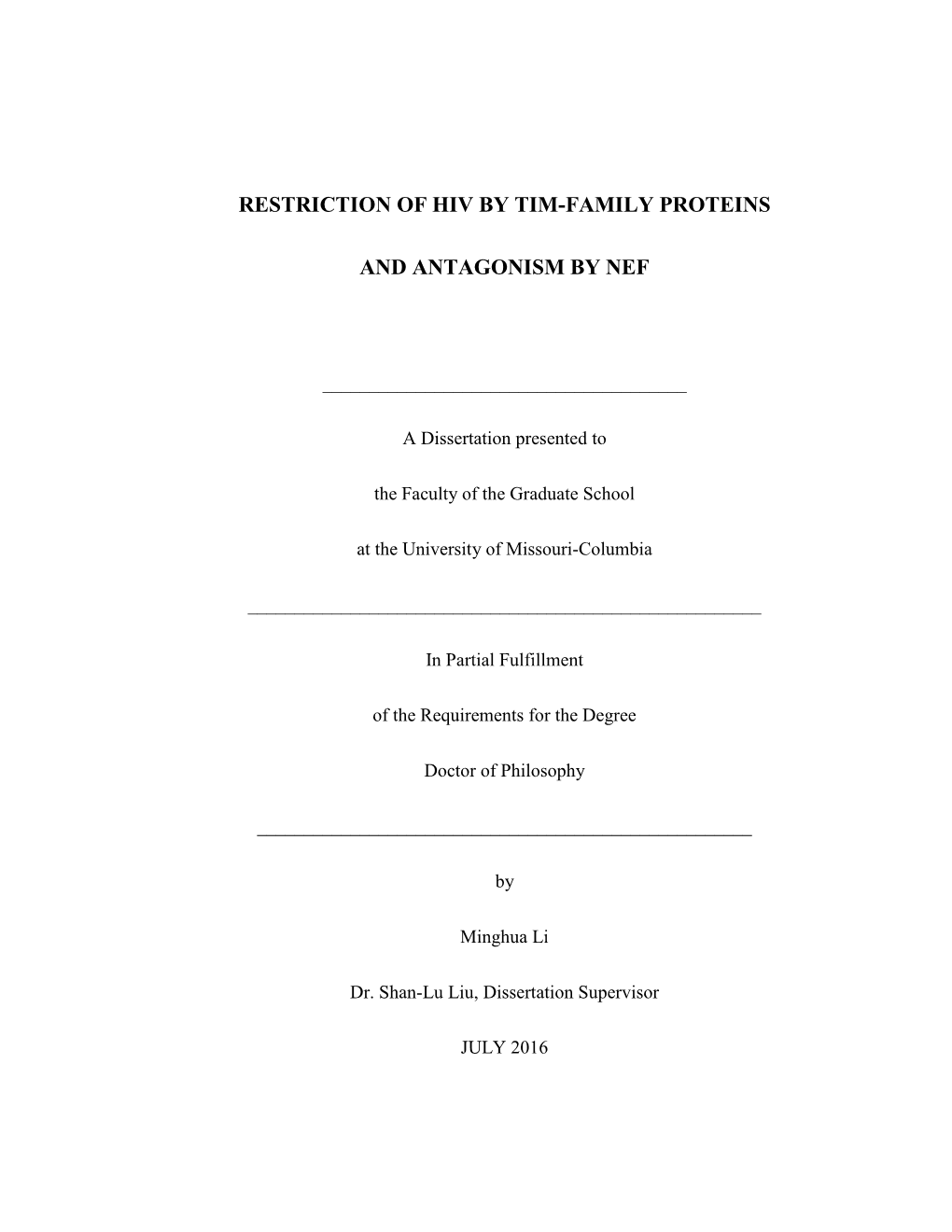
Load more
Recommended publications
-
The Nef-Infectivity Enigma: Mechanisms of Enhanced Lentiviral Infection Jolien Vermeire§, Griet Vanbillemont§, Wojciech Witkowski and Bruno Verhasselt*
View metadata, citation and similar papers at core.ac.uk brought to you by CORE provided by PubMed Central 474 Current HIV Research, 2011, 9, 474-489 The Nef-Infectivity Enigma: Mechanisms of Enhanced Lentiviral Infection Jolien Vermeire§, Griet Vanbillemont§, Wojciech Witkowski and Bruno Verhasselt* Department of Clinical Chemistry, Microbiology, and Immunology, Ghent University, Belgium Abstract: The Nef protein is an essential factor for lentiviral pathogenesis in humans and other simians. Despite a multitude of functions attributed to this protein, the exact role of Nef in disease progression remains unclear. One of its most intriguing functions is the ability of Nef to enhance the infectivity of viral particles. In this review we will discuss current insights in the mechanism of this well-known, yet poorly understood Nef effect. We will elaborate on effects of Nef, on both virion biogenesis and the early stage of the cellular infection, that might be involved in infectivity enhancement. In addition, we provide an overview of different HIV-1 Nef domains important for optimal infectivity and briefly discuss some possible sources of the frequent discrepancies in the field. Hereby we aim to contribute to a better understanding of this highly conserved and therapeutically attractive Nef function. Keywords: Nef, HIV, infectivity, viral replication, mutation analysis, envelope protein, cholesterol, proteasome. 1. INTRODUCTION 2. THE MULTIFACETED NEF PROTEIN Despite the globally declining number of new human As early as 1991, infections of rhesus monkeys with nef- immunodeficiency virus (HIV) infections [1] and the hopeful deleted simian immunodeficiency virus (SIV) revealed a observation that cure from HIV infection does not seem dramatic reduction of viral loads and disease progression in impossible [2], the HIV pandemic still remains a very absence of nef [6]. -
HIV-1 Rev Downregulates Tat Expression and Viral Replication Via Modulation of NAD(P)H:Quinine Oxidoreductase 1 (NQO1)
ARTICLE Received 25 Jul 2014 | Accepted 22 Apr 2015 | Published 10 Jun 2015 DOI: 10.1038/ncomms8244 HIV-1 Rev downregulates Tat expression and viral replication via modulation of NAD(P)H:quinine oxidoreductase 1 (NQO1) Sneh Lata1,*, Amjad Ali2,*, Vikas Sood1,2,w, Rameez Raja2 & Akhil C. Banerjea2 HIV-1 gene expression and replication largely depend on the regulatory proteins Tat and Rev, but it is unclear how the intracellular levels of these viral proteins are regulated after infection. Here we report that HIV-1 Rev causes specific degradation of cytoplasmic Tat, which results in inhibition of HIV-1 replication. The nuclear export signal (NES) region of Rev is crucial for this activity but is not involved in direct interactions with Tat. Rev reduces the levels of ubiquitinated forms of Tat, which have previously been reported to be important for its transcriptional properties. Tat is stabilized in the presence of NAD(P)H:quinine oxidoreductase 1 (NQO1), and potent degradation of Tat is induced by dicoumarol, an NQO1 inhibitor. Furthermore, Rev causes specific reduction in the levels of endogenous NQO1. Thus, we propose that Rev is able to induce degradation of Tat indirectly by downregulating NQO1 levels. Our findings have implications in HIV-1 gene expression and latency. 1 Department of Microbiology, University College of Medical Sciences and Guru Teg Bahadur Hospital, Delhi 110095, India. 2 Laboratory of Virology, National Institute of Immunology, New Delhi 110067, India. * These authors contributed equally to this work. w Present address: Translational Health Science and Technology Institute, Faridabad, Haryana 121004, India. Correspondence and requests for materials should be addressed to A.C.B. -
And Giant Guitarfish (Rhynchobatus Djiddensis)
VIRAL DISCOVERY IN BLUEGILL SUNFISH (LEPOMIS MACROCHIRUS) AND GIANT GUITARFISH (RHYNCHOBATUS DJIDDENSIS) BY HISTOPATHOLOGY EVALUATION, METAGENOMIC ANALYSIS AND NEXT GENERATION SEQUENCING by JENNIFER ANNE DILL (Under the Direction of Alvin Camus) ABSTRACT The rapid growth of aquaculture production and international trade in live fish has led to the emergence of many new diseases. The introduction of novel disease agents can result in significant economic losses, as well as threats to vulnerable wild fish populations. Losses are often exacerbated by a lack of agent identification, delay in the development of diagnostic tools and poor knowledge of host range and susceptibility. Examples in bluegill sunfish (Lepomis macrochirus) and the giant guitarfish (Rhynchobatus djiddensis) will be discussed here. Bluegill are popular freshwater game fish, native to eastern North America, living in shallow lakes, ponds, and slow moving waterways. Bluegill experiencing epizootics of proliferative lip and skin lesions, characterized by epidermal hyperplasia, papillomas, and rarely squamous cell carcinoma, were investigated in two isolated poopulations. Next generation genomic sequencing revealed partial DNA sequences of an endogenous retrovirus and the entire circular genome of a novel hepadnavirus. Giant Guitarfish, a rajiform elasmobranch listed as ‘vulnerable’ on the IUCN Red List, are found in the tropical Western Indian Ocean. Proliferative skin lesions were observed on the ventrum and caudal fin of a juvenile male quarantined at a public aquarium following international shipment. Histologically, lesions consisted of papillomatous epidermal hyperplasia with myriad large, amphophilic, intranuclear inclusions. Deep sequencing and metagenomic analysis produced the complete genomes of two novel DNA viruses, a typical polyomavirus and a second unclassified virus with a 20 kb genome tentatively named Colossomavirus. -
Symposium on Viral Membrane Proteins
Viral Membrane Proteins ‐ Shanghai 2011 交叉学科论坛 Symposium for Advanced Studies 第二十七期:病毒离子通道蛋白的结构与功能研讨会 Symposium on Viral Membrane Proteins 主办单位:中国科学院上海交叉学科研究中心 承办单位:上海巴斯德研究所 1 Viral Membrane Proteins ‐ Shanghai 2011 Symposium on Viral Membrane Proteins Shanghai Institute for Advanced Studies, CAS Institut Pasteur of Shanghai,CAS 30.11. – 2.12 2011 Shanghai, China 2 Viral Membrane Proteins ‐ Shanghai 2011 Schedule: Wednesday, 30th of November 2011 Morning Arrival Thursday, 1st of December 2011 8:00 Arrival 9:00 Welcome Bing Sun, Co-Director, Pasteur Institute Shanghai 9: 10 – 9:35 Bing Sun, Pasteur Institute Shanghai Ion channel study and drug target fuction research of coronavirus 3a like protein. 9:35 – 10:00 Tim Cross, Tallahassee, USA The proton conducting mechanism and structure of M2 proton channel in lipid bilayers. 10:00 – 10:25 Shy Arkin, Jerusalem, IL A backbone structure of SARS Coronavirus E protein based on Isotope edited FTIR, X-ray reflectivity and biochemical analysis. 10:20 – 10:45 Coffee Break 10:45 – 11:10 Rainer Fink, Heidelberg, DE Elektromechanical coupling in muscle: a viral target? 11:10 – 11:35 Yechiel Shai, Rehovot, IL The interplay between HIV1 fusion peptide, the transmembrane domain and the T-cell receptor in immunosuppression. 11:35 – 12:00 Christoph Cremer, Mainz and Heidelberg University, DE Super-resolution Fluorescence imaging of cellular and viral nanostructures. 12:00 – 13:30 Lunch Break 3 Viral Membrane Proteins ‐ Shanghai 2011 13:30 – 13:55 Jung-Hsin Lin, National Taiwan University Robust Scoring Functions for Protein-Ligand Interactions with Quantum Chemical Charge Models. 13:55 – 14:20 Martin Ulmschneider, Irvine, USA Towards in-silico assembly of viral channels: the trials and tribulations of Influenza M2 tetramerization. -
Opportunistic Intruders: How Viruses Orchestrate ER Functions to Infect Cells
REVIEWS Opportunistic intruders: how viruses orchestrate ER functions to infect cells Madhu Sudhan Ravindran*, Parikshit Bagchi*, Corey Nathaniel Cunningham and Billy Tsai Abstract | Viruses subvert the functions of their host cells to replicate and form new viral progeny. The endoplasmic reticulum (ER) has been identified as a central organelle that governs the intracellular interplay between viruses and hosts. In this Review, we analyse how viruses from vastly different families converge on this unique intracellular organelle during infection, co‑opting some of the endogenous functions of the ER to promote distinct steps of the viral life cycle from entry and replication to assembly and egress. The ER can act as the common denominator during infection for diverse virus families, thereby providing a shared principle that underlies the apparent complexity of relationships between viruses and host cells. As a plethora of information illuminating the molecular and cellular basis of virus–ER interactions has become available, these insights may lead to the development of crucial therapeutic agents. Morphogenesis Viruses have evolved sophisticated strategies to establish The ER is a membranous system consisting of the The process by which a virus infection. Some viruses bind to cellular receptors and outer nuclear envelope that is contiguous with an intri‑ particle changes its shape and initiate entry, whereas others hijack cellular factors that cate network of tubules and sheets1, which are shaped by structure. disassemble the virus particle to facilitate entry. After resident factors in the ER2–4. The morphology of the ER SEC61 translocation delivering the viral genetic material into the host cell and is highly dynamic and experiences constant structural channel the translation of the viral genes, the resulting proteins rearrangements, enabling the ER to carry out a myriad An endoplasmic reticulum either become part of a new virus particle (or particles) of functions5. -
Ebola Virus VP40 Real-Time RT-PCR Assay for Use Under an Emergency Use Authorization Only
Ebola Virus VP40 Real-Time RT-PCR Assay Centers for Disease Control and Prevention For Use Under an Emergency Use Authorization Only Instructions for Use January 2016 Page 0 of 50 Table of Contents Introduction .................................................................................................................................... 2 Specimens ....................................................................................................................................... 3 Equipment and Consumables ........................................................................................................ 3 Quality Control ............................................................................................................................... 5 Nucleic Acid Extraction ................................................................................................................. 7 Testing Algorithm .......................................................................................................................... 8 rRT-PCR Assay .............................................................................................................................. 9 Interpreting Test Results .............................................................................................................. 14 Overall Test Interpretation and Reporting Instructions ............................................................. 18 Assay Limitations, Warnings and Precautions .......................................................................... -
WHO Expert Committee on Biological Standardization: Sixty-Eighth Report (WHO Technical Report Series, No
This report presents the recommendations of a WHO Expert Committee commissioned to coordinate activities leading to the 1011 adoption of international recommendations for the production WHO Technical Report Series and control of vaccines and other biological substances, and the establishment of international biological reference materials. 1011 Following a brief introduction, the report summarizes a number WHO of general issues brought to the attention of the Committee. The next part of the report, of particular relevance to manufacturers Expert on Biological Standardization Committee and national regulatory authorities, outlines the discussions held on the development and adoption of new and revised WHO Recommendations, Guidelines and guidance documents. Following these discussions, WHO Guidelines on the quality, safety and efficacy of Ebola vaccines, and WHO Guidelines on procedures and data requirements for changes to approved biotherapeutic products were adopted on the recommendation of the Committee. In addition, the following two WHO guidance documents on the WHO prequalification of in vitro diagnostic medical devices were also adopted: (a) Technical Specifications Series (TSS) for WHO Prequalification – WHO Expert Committee Diagnostic Assessment: Human immunodeficiency virus (HIV) rapid diagnostic tests for professional use and/or self- on Biological testing; and (b) Technical Guidance Series (TGS) for WHO Prequalification – Diagnostic Assessment: Establishing stability of in vitro diagnostic medical devices. Standardization Subsequent -
Virus-Host Interaction: the Multifaceted Roles of Ifitms And
Virus-Host Interaction: The Multifaceted Roles of IFITMs and LY6E in HIV Infection DISSERTATION Presented in Partial Fulfillment of the Requirements for the Degree Doctor of Philosophy in the Graduate School of The Ohio State University By Jingyou Yu Graduate Program in Comparative and Veterinary Medicine The Ohio State University 2018 Dissertation Committee: Shan-Lu Liu, MD, PhD, Advisor Patrick L. Green, PhD Jianrong Li, DVM., PhD Jesse J. Kwiek, PhD Copyrighted by Jingyou Yu 2018 Abstract With over 1.8 million newly infected people each year, the worldwide HIV-1 epidemic remains an imperative challenge for public health. Recent work has demonstrated that type I interferons (IFNs) efficiently suppress HIV infection through induction of hundreds of interferon stimulated genes (ISGs). These ISGs target distinct infection stages of invading pathogens and shape innate immunity. Among these, interferon induced transmembrane proteins (IFITMs) and lymphocyte antigen 6 complex, locus E (LY6E) have been shown to differentially modulate viral infections. However, their effects on HIV are not fully understood. In my thesis work, I provided evidence in Chapter 2 showing that IFITM proteins, particularly IFITM2 and IFITM3, specifically antagonize the HIV-1 envelope glycoprotein (Env), thereby inhibiting viral infection. IFITM proteins interacted with HIV-1 Env in viral producer cells, leading to impaired Env processing and virion incorporation. Notably, the level of IFITM incorporation into HIV-1 virions did not strictly correlate with the extent of inhibition. Prolonged passage of HIV-1 in IFITM-expressing T lymphocytes led to emergence of Env mutants that overcome IFITM restriction. The ability of IFITMs to inhibit cell-to-cell infection can be extended to HIV-1 primary isolates, HIV-2 and SIVs; however, the extent of inhibition appeared to be virus- strain dependent. -
A Novel Ebola Virus VP40 Matrix Protein-Based Screening for Identification of Novel Candidate Medical Countermeasures
viruses Communication A Novel Ebola Virus VP40 Matrix Protein-Based Screening for Identification of Novel Candidate Medical Countermeasures Ryan P. Bennett 1,† , Courtney L. Finch 2,† , Elena N. Postnikova 2 , Ryan A. Stewart 1, Yingyun Cai 2 , Shuiqing Yu 2 , Janie Liang 2, Julie Dyall 2 , Jason D. Salter 1 , Harold C. Smith 1,* and Jens H. Kuhn 2,* 1 OyaGen, Inc., 77 Ridgeland Road, Rochester, NY 14623, USA; [email protected] (R.P.B.); [email protected] (R.A.S.); [email protected] (J.D.S.) 2 NIH/NIAID/DCR/Integrated Research Facility at Fort Detrick (IRF-Frederick), Frederick, MD 21702, USA; courtney.fi[email protected] (C.L.F.); [email protected] (E.N.P.); [email protected] (Y.C.); [email protected] (S.Y.); [email protected] (J.L.); [email protected] (J.D.) * Correspondence: [email protected] (H.C.S.); [email protected] (J.H.K.); Tel.: +1-585-697-4351 (H.C.S.); +1-301-631-7245 (J.H.K.) † These authors contributed equally to this work. Abstract: Filoviruses, such as Ebola virus and Marburg virus, are of significant human health concern. From 2013 to 2016, Ebola virus caused 11,323 fatalities in Western Africa. Since 2018, two Ebola virus disease outbreaks in the Democratic Republic of the Congo resulted in 2354 fatalities. Although there is progress in medical countermeasure (MCM) development (in particular, vaccines and antibody- based therapeutics), the need for efficacious small-molecule therapeutics remains unmet. Here we describe a novel high-throughput screening assay to identify inhibitors of Ebola virus VP40 matrix protein association with viral particle assembly sites on the interior of the host cell plasma membrane. -
Molecular Analysis of the Complete Genome of a Simian Foamy Virus Infecting Hylobates Pileatus (Pileated Gibbon) Reveals Ancient Co-Evolution with Lesser Apes
viruses Article Molecular Analysis of the Complete Genome of a Simian Foamy Virus Infecting Hylobates pileatus (pileated gibbon) Reveals Ancient Co-Evolution with Lesser Apes Anupama Shankar 1, Samuel D. Sibley 2, Tony L. Goldberg 2 and William M. Switzer 1,* 1 Laboratory Branch, Division of HIV/AIDS Prevention, Center for Disease Control and Prevention, Atlanta, GA 30329, USA 2 Department of Pathobiological Sciences, School of Veterinary Medicine, University of Wisconsin-Madison, Madison, WI 53706, USA * Correspondence: [email protected]; Tel.: +1-404-639-2019 Received: 1 April 2019; Accepted: 30 June 2019; Published: 3 July 2019 Abstract: Foamy viruses (FVs) are complex retroviruses present in many mammals, including nonhuman primates, where they are called simian foamy viruses (SFVs). SFVs can zoonotically infect humans, but very few complete SFV genomes are available, hampering the design of diagnostic assays. Gibbons are lesser apes widespread across Southeast Asia that can be infected with SFV, but only two partial SFV sequences are currently available. We used a metagenomics approach with next-generation sequencing of nucleic acid extracted from the cell culture of a blood specimen from a lesser ape, the pileated gibbon (Hylobates pileatus), to obtain the complete SFVhpi_SAM106 genome. We used Bayesian analysis to co-infer phylogenetic relationships and divergence dates. SFVhpi_SAM106 is ancestral to other ape SFVs with a divergence date of ~20.6 million years ago, reflecting ancient co-evolution of the host and SFVhpi_SAM106. Analysis of the complete SFVhpi_SAM106 genome shows that it has the same genetic architecture as other SFVs but has the longest recorded genome (13,885-nt) due to a longer long terminal repeat region (2,071 bp). -
Lentivirus and Lentiviral Vectors Fact Sheet
Lentivirus and Lentiviral Vectors Family: Retroviridae Genus: Lentivirus Enveloped Size: ~ 80 - 120 nm in diameter Genome: Two copies of positive-sense ssRNA inside a conical capsid Risk Group: 2 Lentivirus Characteristics Lentivirus (lente-, latin for “slow”) is a group of retroviruses characterized for a long incubation period. They are classified into five serogroups according to the vertebrate hosts they infect: bovine, equine, feline, ovine/caprine and primate. Some examples of lentiviruses are Human (HIV), Simian (SIV) and Feline (FIV) Immunodeficiency Viruses. Lentiviruses can deliver large amounts of genetic information into the DNA of host cells and can integrate in both dividing and non- dividing cells. The viral genome is passed onto daughter cells during division, making it one of the most efficient gene delivery vectors. Most lentiviral vectors are based on the Human Immunodeficiency Virus (HIV), which will be used as a model of lentiviral vector in this fact sheet. Structure of the HIV Virus The structure of HIV is different from that of other retroviruses. HIV is roughly spherical with a diameter of ~120 nm. HIV is composed of two copies of positive ssRNA that code for nine genes enclosed by a conical capsid containing 2,000 copies of the p24 protein. The ssRNA is tightly bound to nucleocapsid proteins, p7, and enzymes needed for the development of the virion: reverse transcriptase (RT), proteases (PR), ribonuclease and integrase (IN). A matrix composed of p17 surrounds the capsid ensuring the integrity of the virion. This, in turn, is surrounded by an envelope composed of two layers of phospholipids taken from the membrane of a human cell when a newly formed virus particle buds from the cell. -
10 International Foamy Virus Conference 2014
10th INTERNATIONAL FOAMY VIRUS CONFERENCE 2014 June 24‐25, 2014 National Veterinary Research Institute, Pulawy, Poland Organized by: National Veterinary Research Institute Committee of Veterinary Sciences Polish Academy of Sciences Topics include: Foamy Virus Infection and Zoonotic Aspects, Foamy Virus Restriction and Immunity, Foamy Virus Biology and Gene Therapy Vectors http://www.piwet.pulawy.pl/ifvc/ Organizers Jacek Kuźmak and Magdalena Materniak Scientific Advisors Martin Löchelt and Axel Rethwilm Sponsors Support for the meeting was provided by the Committee of Veterinary Sciences Polish Academy of Sciences, ROCHE, Thermo Fisher Scientific and EURx Abstract book was issued thanks to the support provided by Committee of Veterinary Sciences Polish Academy of Sciences 2 Program Overview Monday, June 23rd, 2014 19 00 – 20 00 Registration 20 30 – open end Welcome Reception Tuesday, June 24th, 2014 9 00 – 9 30 Registration 9 30 – 9 45 Welcome 9 45 – 10 15 Arifa Khan (FDA, Bethesda, US) - Simian foamy virus infection in natural host species 10 15 – 10 45 Antoine Gessain (Institute Pasteur, Paris, France) – Zoonotic human infection by simian foamy viruses 10 45 – 11 20 Session 1 on Foamy Virus Infection and Zoonotic Aspects Chairs: Arifa S. Khan and Antoine Gessain 11 20 – 11 45 Coffee break 11 45 – 12 30 Session 1 on Foamy Virus Infection and Zoonotic Aspects Chairs: Arifa S. Khan and Antoine Gessain 12 30 – 13 00 Aris Katzourakis (Oxford University, UK - Mammalian genomes, their endogenous viral elements, and the evolutionary history