Intermodal Group Velocity Engineering for Broadband Nonlinear Optics
Total Page:16
File Type:pdf, Size:1020Kb
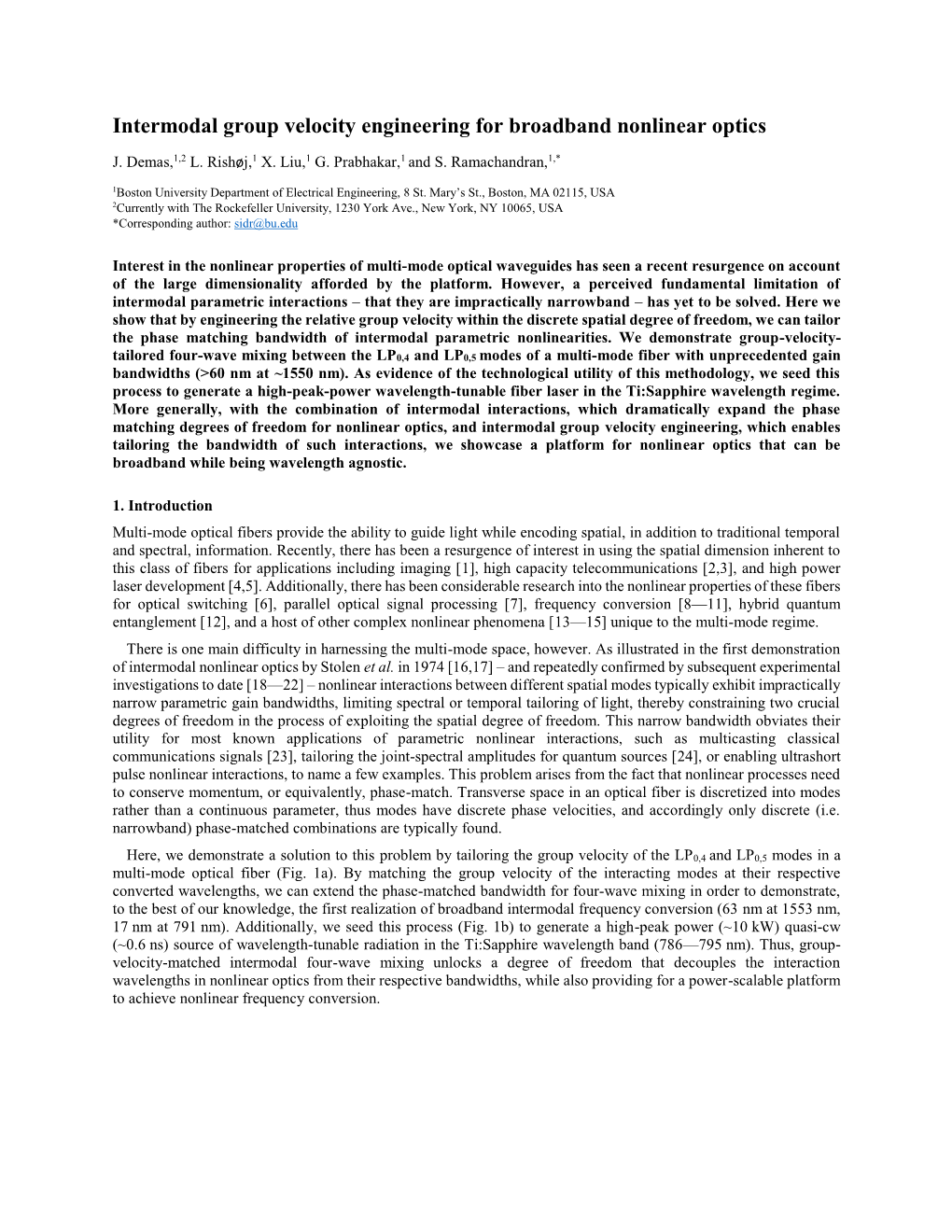
Load more
Recommended publications
-
Group Velocity
Particle Waves and Group Velocity Particles with known energy Consider a particle with mass m, traveling in the +x direction and known velocity 1 vo and energy Eo = mvo . The wavefunction that represents this particle is: 2 !(x,t) = Ce jkxe" j#t [1] where C is a constant and 2! 1 ko = = 2mEo [2] "o ! Eo !o = 2"#o = [3] ! 2 The envelope !(x,t) of this wavefunction is 2 2 !(x,t) = C , which is a constant. This means that when a particle’s energy is known exactly, it’s position is completely unknown. This is consistent with the Heisenberg Uncertainty principle. Even though the magnitude of this wave function is a constant with respect to both position and time, its phase is not. As with any type of wavefunction, the phase velocity vp of this wavefunction is: ! E / ! v v = = o = E / 2m = v2 / 4 = o [4] p k 1 o o 2 2mEo ! At first glance, this result seems wrong, since we started with the assumption that the particle is moving at velocity vo. However, only the magnitude of a wavefunction contains measurable information, so there is no reason to believe that its phase velocity is the same as the particle’s velocity. Particles with uncertain energy A more realistic situation is when there is at least some uncertainly about the particle’s energy and momentum. For real situations, a particle’s energy will be known to lie only within some band of uncertainly. This can be handled by assuming that the particle’s wavefunction is the superposition of a range of constant-energy wavefunctions: jkn x # j$nt !(x,t) = "Cn (e e ) [5] n Here, each value of kn and ωn correspond to energy En, and Cn is the probability that the particle has energy En. -
Photons That Travel in Free Space Slower Than the Speed of Light Authors
Title: Photons that travel in free space slower than the speed of light Authors: Daniel Giovannini1†, Jacquiline Romero1†, Václav Potoček1, Gergely Ferenczi1, Fiona Speirits1, Stephen M. Barnett1, Daniele Faccio2, Miles J. Padgett1* Affiliations: 1 School of Physics and Astronomy, SUPA, University of Glasgow, Glasgow G12 8QQ, UK 2 School of Engineering and Physical Sciences, SUPA, Heriot-Watt University, Edinburgh EH14 4AS, UK † These authors contributed equally to this work. * Correspondence to: [email protected] Abstract: That the speed of light in free space is constant is a cornerstone of modern physics. However, light beams have finite transverse size, which leads to a modification of their wavevectors resulting in a change to their phase and group velocities. We study the group velocity of single photons by measuring a change in their arrival time that results from changing the beam’s transverse spatial structure. Using time-correlated photon pairs we show a reduction of the group velocity of photons in both a Bessel beam and photons in a focused Gaussian beam. In both cases, the delay is several microns over a propagation distance of the order of 1 m. Our work highlights that, even in free space, the invariance of the speed of light only applies to plane waves. Introducing spatial structure to an optical beam, even for a single photon, reduces the group velocity of the light by a readily measurable amount. One sentence summary: The group velocity of light in free space is reduced by controlling the transverse spatial structure of the light beam. Main text The speed of light is trivially given as �/�, where � is the speed of light in free space and � is the refractive index of the medium. -
Theory and Application of SBS-Based Group Velocity Manipulation in Optical Fiber
Theory and Application of SBS-based Group Velocity Manipulation in Optical Fiber by Yunhui Zhu Department of Physics Duke University Date: Approved: Daniel J. Gauthier, Advisor Henry Greenside Jungsang Kim Haiyan Gao David Skatrud Dissertation submitted in partial fulfillment of the requirements for the degree of Doctor of Philosophy in the Department of Physics in the Graduate School of Duke University 2013 ABSTRACT (Physics) Theory and Application of SBS-based Group Velocity Manipulation in Optical Fiber by Yunhui Zhu Department of Physics Duke University Date: Approved: Daniel J. Gauthier, Advisor Henry Greenside Jungsang Kim Haiyan Gao David Skatrud An abstract of a dissertation submitted in partial fulfillment of the requirements for the degree of Doctor of Philosophy in the Department of Physics in the Graduate School of Duke University 2013 Copyright c 2013 by Yunhui Zhu All rights reserved Abstract All-optical devices have attracted many research interests due to their ultimately low heat dissipation compared to conventional devices based on electric-optical conver- sion. With recent advances in nonlinear optics, it is now possible to design the optical properties of a medium via all-optical nonlinear effects in a table-top device or even on a chip. In this thesis, I realize all-optical control of the optical group velocity using the nonlinear process of stimulated Brillouin scattering (SBS) in optical fibers. The SBS- based techniques generally require very low pump power and offer a wide transparent window and a large tunable range. Moreover, my invention of the arbitrary SBS res- onance tailoring technique enables engineering of the optical properties to optimize desired function performance, which has made the SBS techniques particularly widely adapted for various applications. -
Phase and Group Velocities of Surface Waves in Left-Handed Material Waveguide Structures
Optica Applicata, Vol. XLVII, No. 2, 2017 DOI: 10.5277/oa170213 Phase and group velocities of surface waves in left-handed material waveguide structures SOFYAN A. TAYA*, KHITAM Y. ELWASIFE, IBRAHIM M. QADOURA Physics Department, Islamic University of Gaza, Gaza, Palestine *Corresponding author: [email protected] We assume a three-layer waveguide structure consisting of a dielectric core layer embedded between two left-handed material claddings. The phase and group velocities of surface waves supported by the waveguide structure are investigated. Many interesting features were observed such as normal dispersion behavior in which the effective index increases with the increase in the propagating wave frequency. The phase velocity shows a strong dependence on the wave frequency and decreases with increasing the frequency. It can be enhanced with the increase in the guiding layer thickness. The group velocity peaks at some value of the normalized frequency and then decays. Keywords: slab waveguide, phase velocity, group velocity, left-handed materials. 1. Introduction Materials with negative electric permittivity ε and magnetic permeability μ are artifi- cially fabricated metamaterials having certain peculiar properties which cannot be found in natural materials. The unusual properties include negative refractive index, reversed Goos–Hänchen shift, reversed Doppler shift, and reversed Cherenkov radiation. These materials are known as left-handed materials (LHMs) since the electric field, magnetic field, and the wavevector form a left-handed set. VESELAGO was the first who investi- gated the propagation of electromagnetic waves in such media and predicted a number of unusual properties [1]. Till now, LHMs have been realized successfully in micro- waves, terahertz waves and optical waves. -
Chapter 3 Wave Properties of Particles
Chapter 3 Wave Properties of Particles Overview of Chapter 3 Einstein introduced us to the particle properties of waves in 1905 (photoelectric effect). Compton scattering of x-rays by electrons (which we skipped in Chapter 2) confirmed Einstein's theories. You ought to ask "Is there a converse?" Do particles have wave properties? De Broglie postulated wave properties of particles in his thesis in 1924, based partly on the idea that if waves can behave like particles, then particles should be able to behave like waves. Werner Heisenberg and a little later Erwin Schrödinger developed theories based on the wave properties of particles. In 1927, Davisson and Germer confirmed the wave properties of particles by diffracting electrons from a nickel single crystal. 3.1 de Broglie Waves Recall that a photon has energy E=hf, momentum p=hf/c=h/, and a wavelength =h/p. De Broglie postulated that these equations also apply to particles. In particular, a particle of mass m moving with velocity v has a de Broglie wavelength of h λ = . mv where m is the relativistic mass m m = 0 . 1-v22/ c In other words, it may be necessary to use the relativistic momentum in =h/mv=h/p. In order for us to observe a particle's wave properties, the de Broglie wavelength must be comparable to something the particle interacts with; e.g. the spacing of a slit or a double slit, or the spacing between periodic arrays of atoms in crystals. The example on page 92 shows how it is "appropriate" to describe an electron in an atom by its wavelength, but not a golf ball in flight. -
Module 2 – Linear Fiber Propagation
Module 2 – Linear Fiber Propagation Dr. E. M. Wright Professor, College of Optics, University of Arizona Dr. E. M. Wright is a Professor of Optical Sciences and Physic sin the University of Arizona. Research activity within Dr. Wright's group is centered on the theory and simulation of propagation in nonlinear optical media including optical fibers, integrated optics, and bulk media. Active areas of current research include nonlinear propagation of light strings in the atmosphere, and the propagation of novel laser fields for applications in optical manipulation and trapping of particles, and quantum nonlinear optics. Email: [email protected] Introduction The goal of modules 2-5 is to build an understanding of nonlinear fiber optics and in particular temporal optical solitons. To accomplish this goal we shall refer to material from module 1 of the graduate super-course, Electromagnetic Wave Propagation, as well as pointing to the connections with several undergraduate (UG) modules as we go along. We start in module 2 with a treatment of linear pulse propagation in fibers that will facilitate the transition to nonlinear propagation and also establish notation, and in module 3 we shall discuss numerical pulse propagation both as a simulation tool and an aid to understanding. Nonlinear fiber properties will be discussed in module 4, and temporal solitons arising from the combination of linear and nonlinear properties in optical fibers shall be treated in module 5. The learning outcomes for this module include • The student will be conversant with the LP modes of optical fibers and how to calculate the properties of the fundamental mode. -
Phase and Group Velocity
PHASEN- UND GRUPPENGESCHWINDIGKEIT VON ULTRASCHALL IN FLÜSSIGKEITEN Phase and group velocity of ultrasonic waves in liquids 1 Goal In this experiment we want to measure phase and group velocity of sound waves in liquids. Consequently, we measure time and space propagation of ultrasonic waves (frequencies above 20 kHz) in water, saline and glycerin. 2 Theory 2.1 Phase Velocity Sound is a temporal and special periodic sequence of positive and negative pressures. Since the changes in the pressure occur parallel to the propagation direction, it is called a longitudinal wave. The pressure function P(x, t) can be described by a cosine function: 22ππ Pxt(,)= A⋅− cos( kxωω t )mit k = und = ,(1) λ T where A is the amplitude of the wave, and k is the wave number and λ is the wavelength. The propagation P(x,t) c velocity CPh is expressed with the help of a reference Ph point (e.g. the maximum).in the space. The wave propagates with the velocity of the points that have the A same phase position in space. Therefore, Cph is called phase velocity. Depending on the frequency ν it is P0 x,t defined as ω c =λν⋅= (2). Ph k The phase velocity (speed of sound) is 331 m/s in the air. The phase velocity reaches around 1500 m/s in the water because of the incompressibility of liquids. It increases even to 6000 m/s in solids. During this process, energy and momentum is transported in a medium without requiring a mass transfer. 1 PHASEN- UND GRUPPENGESCHWINDIGKEIT VON ULTRASCHALL IN FLÜSSIGKEITEN 2.2 Group Velocity P(x,t) A wave packet or wave group consists of a superposition of cG several waves with different frequencies, wavelengths and amplitudes (Not to be confused with interference that arises when superimposing waves with the same frequencies!). -
Effect of Phonon Dispersion on Thermal Conduction Across Si/Ge Interfaces1
Effect of phonon dispersion on thermal conduction across Si/Ge interfaces1 Dhruv Singh Jayathi Y. Murthy* Timothy S. Fisher School of Mechanical Engineering and Birck Nanotechnology Center, Purdue University, West Lafayette, IN -47907, USA ABSTRACT We report finite-volume simulations of the phonon Boltzmann transport equation (BTE) for heat conduction across the heterogeneous interfaces in SiGe superlattices. The diffuse mismatch model incorporating phonon dispersion and polarization is implemented over a wide range of Knudsen numbers. The results indicate that the thermal conductivity of a Si/Ge superlattice is much lower than that of the constitutive bulk materials for superlattice periods in the submicron regime. We report results for effective thermal conductivity of various material volume fractions and superlattice periods. Details of the non-equilibrium energy exchange between optical and acoustic phonons that originate from the mismatch of phonon spectra in silicon and germanium are delineated for the first time. Conditions are identified for which this effect can produce significantly more thermal resistance than that due to boundary scattering of phonons. * Corresponding Author, e-mail: [email protected] 1Paper presented at the 2009 ASME/Pacific Rim Technical Conference and Exhibition on Packaging and Integration of Electronic and Photonic Systems, MEMS and NEMS (InterPACK ‟09), San Francisco, CA, Jul 19-23, 2009. 1 I. INTRODUCTION During the past decade, there has been growing interest in developing nanostructured materials such as composites and superlattices for use in thermoelectrics, thermal interface materials and in macroelectronics [1-5]. It has long been known that the thermal and electrical transport properties of isolated nanostructures such as thin films, nanowires and nanotubes often exhibit significant deviations from their bulk counterparts [6-8]. -
Femtosecond Optics by Tomas Jankauskas
Femtosecond optics by Tomas Jankauskas Since the introduction of the first sub-picosecond lasers in the 1990s, the market for femtosecond optics Chromatic dispersion has grownrapidly. However, it still cannot compete with longer pulse or CW laser markets. In femtosecond Since the optical spectrum of an ultrashort pulse is very applications problems of conventional optics and broad, group velocity plays a key role in understanding coatings are that they either distort the temporal how ultrashort optics work. Group velocity of a wave characteristics of the pulse, or are damaged by high is the velocity with which the overall shape of waves’ peak power of the pulse. To better understand why this amplitudes propagates through space. It would be happens, let’s look at the basics of the ultrashort world. correct to say that group velocity is the velocity with Though the definition sometimes varies, anultrashort which whole broad electromagnetic ultrashort pulse pulse is an electromagnetic pulse with a time duration propagates. For free space where the refractive index of one picosecond (10-12 second) or less. Since ultrashort is equal to one, group velocity is constant for all phenomena are too fast to be directly measured with components of the pulse. electronic devices such events are sometimes referred Optical materials possess a specific quality, the phase to as ultrafast (the meaning, however, is the same). velocity of light inside the material depends on the Pulse length is inversely proportional to the optical frequency (or wavelength), and equivalently the group spectrum of the laser beam therefore ultrashort pulses velocity depends on the frequency. -
The Anatomy of Negative Refraction
Technical article The Anatomy of Negative Refraction Abstract — The mechanism of negative refraction (electromag- netic waves bending the ‘wrong’ way at material interfaces) and the closely related phenomenon of backward waves (phase veloc- ity opposite to group velocity) are examined. It is shown that in periodic dielectric structures, such as metamaterials and photonic crystals, backward waves disappear in the homogenization limit, when the lattice cell size becomes negligible relative to the vacuum wavelength. The paper establishes a lower bound for the cell size of metamaterials capable of supporting backward waves. Introduc- tory material and historical notes are included. IINTRODUCTION Figure 1: Number of ISI papers published on negative refraction. (ISI database query: “negative refraction” OR “negative index” Negative refraction (electromagnetic waves bending the ‘wrong’ material OR “double-negative” material.) way at material interfaces) and the closely related phenomenon of backward waves (phase velocity opposite to group veloc- ity) have become one of the most intriguing areas of research in nanophotonics this century, with thousands of research pa- ² Light propagating from a regular medium into a double- pers published and a number of books and review papers readily negative material bends “the wrong way" (Fig. 2). In Snell’s available: P.W. Milonni [20], G.V.Eleftheriades & K.G. Balmain law, this corresponds to a negative index of refraction. (eds.) [7], S.A. Ramakrishna [33], J.B. Pendry & D.R. Smith [31], and others. The annual numbers of ISI publications (Fig. 1) ² A slab with ² = ¡1, ¹ = ¡1 in air acts as an unusual lens 2 illustrate the rapidly rising interest in this subject. -
Negative Group Velocity Kirk T
Negative Group Velocity Kirk T. McDonald Joseph Henry Laboratories, Princeton University, Princeton, NJ 08544 (July 23, 2000; updated May 13, 2016) 1Problem Consider a variant on the physical situation of “slow light” [1, 2] in which two closely spaced spectral lines are now both optically pumped to show that the group velocity can be negative at the central frequency, which leads to apparent superluminal behavior. 1.1 Negative Group Velocity In more detail, consider a classical model of matter in which spectral lines are associated with oscillators.1 In particular, consider a gas of atoms with two closely spaced spectral lines of angular frequencies ω1,2 = ω0 ± Δ/2, where Δ ω0. Each line has the same damping constant (and spectral width) γ. Ordinarily, the gas would exhibit strong absorption of light in the vicinity of the spectral lines. But suppose that lasers of frequencies ω1 and ω2 pump the both oscillators into inverted populations. This can be described classically by assigning negative oscillator strengths to these oscillators.2 Deduce an expression for the group velocity vg(ω0) of a pulse of light centered on frequency ω0 in terms of the (univalent) plasma frequency ωp of the medium, given by 4πNe2 ω2 = , (1) p m where N is the number density of atoms, and e and m are the charge and mass of an electron. Give a condition on the line separation Δ compared to the line width γ such that the group velocity vg(ω0) is negative. In a recent experiment by Wang et al. [4], a group velocity of vg = −c/310, where c is the speed of light in vacuum, was demonstrated in cesium vapor using a pair of spectral lines with separation Δ/2π ≈ 2 MHz and linewidth γ/2π ≈ 0.8MHz. -
Ωωω) of the Wave As a Function of Physical Parameters And/Or the Wave Number
ESCI 485 – Air/sea Interaction Lesson 4 – Waves Dr. DeCaria GENERAL WAVE PROPERTIES Each type of wave has its own dispersion characteristics, or dispersion relation . ο The dispersion relation is an equation that gives the frequency ( ωωω) of the wave as a function of physical parameters and/or the wave number. The speed of an individual wave crest is called the phase speed , and is given as c p ≡ ω k The energy of the wave propagates at the group velocity . The group velocity is not necessarily the same as the phase speed. The group velocity is found by ∂ω c ≡ . g ∂k If the phase speed of a wave doesn’t depend on wave number then the wave is called non-dispersive . In this case it turns out that cg = cp. If the phase speed is a function of wave number then the waves are dispersive . DISPERSION CHARACTERISTICS OF CAPILLARY WAVES The dispersion relation for surface capillary/gravity waves is ω= ±(kg + k3 σ ρ ) tanh kH where σσσ is the surface tension. The surface tension term is only important for the shortest wavelengths (on the order of a few centimeters or less). ο For wavelengths smaller than around 1.7 cm only surface tension is important and the waves are pure capillary waves. The dispersion relation for pure capillary waves is ω = ± k 3 ()σ ρ tanh kH . Capillary waves are anomalously dispersive, meaning that the group velocity is larger than the phase speed and that shorter wavelengths are faster than longer wavelengths. Capillary waves attenuate very quickly due to molecular viscosity.