Non-Canonical Replication Initiation: You're Fired!
Total Page:16
File Type:pdf, Size:1020Kb
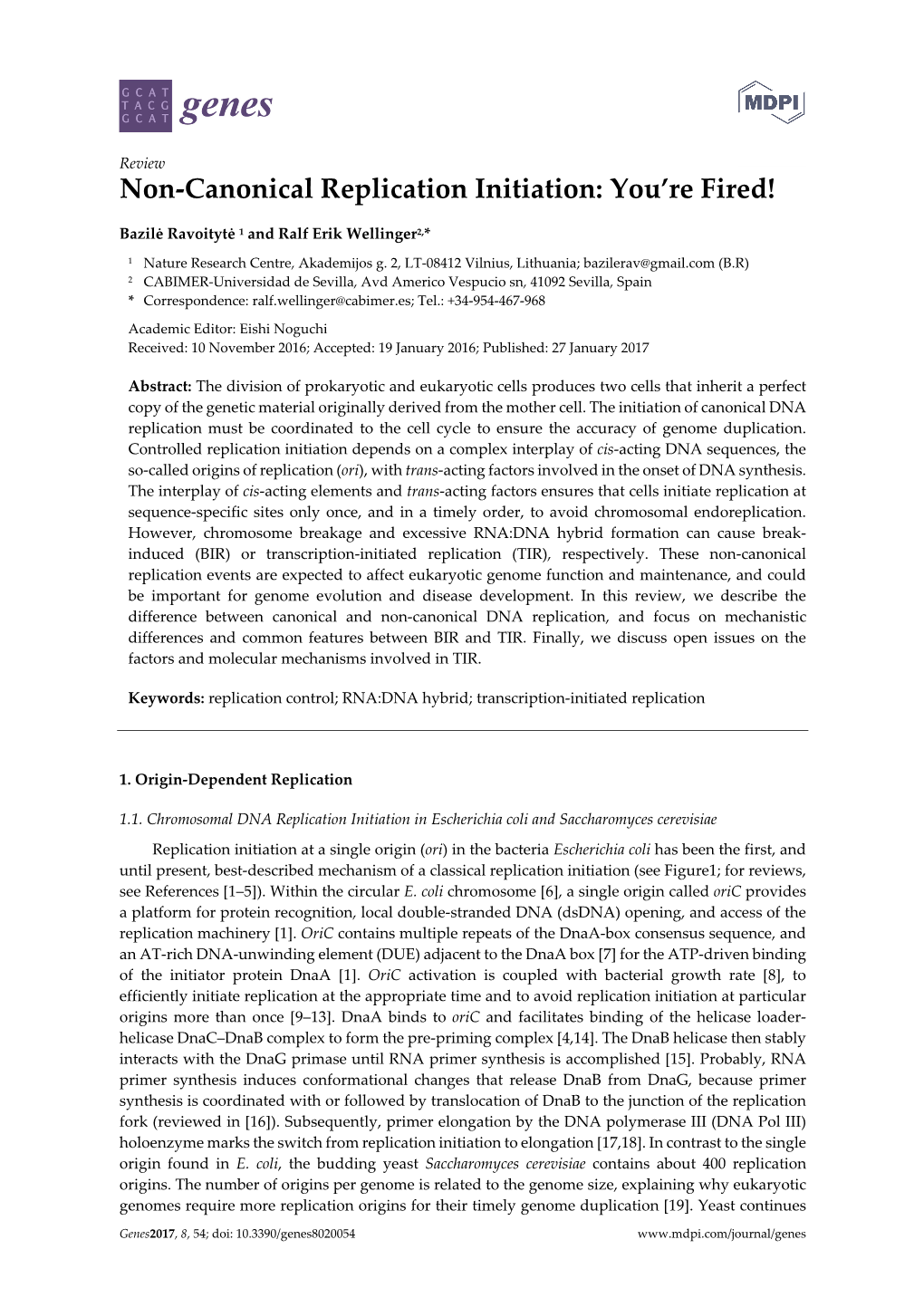
Load more
Recommended publications
-
Replisome Assembly at Oric, the Replication Origin of E. Coli, Reveals an Explanation for Initiation Sites Outside an Origin
Molecular Cell, Vol. 4, 541±553, October, 1999, Copyright 1999 by Cell Press Replisome Assembly at oriC, the Replication Origin of E. coli, Reveals an Explanation for Initiation Sites outside an Origin Linhua Fang,*§ Megan J. Davey,² and Mike O'Donnell²³ have not been addressed. For example, is the local un- *Microbiology Department winding sufficiently large for two helicases to assemble Joan and Sanford I. Weill Graduate School of Medical for bidirectional replication, or does one helicase need Sciences of Cornell University to enter first and expand the bubble via helicase action New York, New York 10021 to make room for the second helicase? The known rep- ² The Rockefeller University and licative helicases are hexameric and encircle ssDNA. Howard Hughes Medical Institute Which strand does the initial helicase(s) at the origin New York, New York 10021 encircle, and if there are two, how are they positioned relative to one another? Primases generally require at least transient interaction with helicase to function. Can Summary primase function with the helicase(s) directly after heli- case assembly at the origin, or must helicase-catalyzed This study outlines the events downstream of origin DNA unwinding occur prior to RNA primer synthesis? unwinding by DnaA, leading to assembly of two repli- Chromosomal replicases are comprised of a ring-shaped cation forks at the E. coli origin, oriC. We show that protein clamp that encircles DNA, a clamp-loading com- two hexamers of DnaB assemble onto the opposing plex that uses ATP to assemble the clamp around DNA, strands of the resulting bubble, expanding it further, and a DNA polymerase that binds the circular clamp, yet helicase action is not required. -
Initiation of Enzymatic Replication at the Origin of the Escherichia
Proc. Nati. Acad. Sci. USA Vol. 82, pp. 3954-3958, June 1985 Biochemistry Initiation of enzymatic replication at the origin of the Escherichia coli chromosome: Primase as the sole priming enzyme (DNA/orC/plasmids) ARIE VAN DER ENDEt, TANIA A. BAKER, TOHRU OGAWA*, AND ARTHUR KORNBERG Department of Biochemistry, Stanford University School of Medicine, Stanford, CA 94305 Contributed by Arthur Kornberg, January 28, 1985 ABSTRACT The enzymatic replication of plasmids con- MATERIALS AND METHODS taining the unique (245 base pair) origin of the Escherichia coli chromosome (oriC) can be initiated with any of three enzyme DNAs and Reagents. pCM959 (4) was a gift from M. Meijer priming systems: primase alone, RNA polymerase alone, or (University of Amsterdam, The Netherlands); pTOA7 (T. both combined (Ogawa, T., Baker, T. A., van der Ende, A. & Ogawa) was constructed by inserting the Hae II-Acc I Kornberg, A. (1985) Proc. Natl. Acad. Sci. USA 82, oriC-containing fragment from M13oriC26 (7) via EcoRI 3562-3566). At certain levels of auxiliary proteins linkers into EcoRI-cleaved pMAPCdSG10, a deletion deriva- (topoisomerase I, protein HU, and RNase H), the solo primase tive of pBR327 (W. A. Segraves, personal communication); system is efficient and responsible for priming synthesis of all pSY317, M13oriC26, M13oriC2LB5, and M13AE101 are DNA strands. Replication of oriC plasmids is here separated described in Table 1 and elsewhere (3, 7). Tricine, creatine into four stages: (i) formation of an isolable, prepriming phosphate, ribo- and deoxyribonucleoside triphosphates complex requiring oriC, dnaA protein, dnaB protein, dnaC (rNTPs and dNTPs) were from Sigma; a-32P-labeled dTTP, protein, gyrase, single-strand binding protein, and ATP; (ii) rATP, rUTP, rGTP, and rCTP (>400 Ci/mmol; 1 Ci = 37 formation of a primed template by primase; (iii) rapid, GBq) were from Amersham. -
Arthur Kornberg Discovered (The First) DNA Polymerase Four
Arthur Kornberg discovered (the first) DNA polymerase Using an “in vitro” system for DNA polymerase activity: 1. Grow E. coli 2. Break open cells 3. Prepare soluble extract 4. Fractionate extract to resolve different proteins from each other; repeat; repeat 5. Search for DNA polymerase activity using an biochemical assay: incorporate radioactive building blocks into DNA chains Four requirements of DNA-templated (DNA-dependent) DNA polymerases • single-stranded template • deoxyribonucleotides with 5’ triphosphate (dNTPs) • magnesium ions • annealed primer with 3’ OH Synthesis ONLY occurs in the 5’-3’ direction Fig 4-1 E. coli DNA polymerase I 5’-3’ polymerase activity Primer has a 3’-OH Incoming dNTP has a 5’ triphosphate Pyrophosphate (PP) is lost when dNMP adds to the chain E. coli DNA polymerase I: 3 separable enzyme activities in 3 protein domains 5’-3’ polymerase + 3’-5’ exonuclease = Klenow fragment N C 5’-3’ exonuclease Fig 4-3 E. coli DNA polymerase I 3’-5’ exonuclease Opposite polarity compared to polymerase: polymerase activity must stop to allow 3’-5’ exonuclease activity No dNTP can be re-made in reversed 3’-5’ direction: dNMP released by hydrolysis of phosphodiester backboneFig 4-4 Proof-reading (editing) of misincorporated 3’ dNMP by the 3’-5’ exonuclease Fidelity is accuracy of template-cognate dNTP selection. It depends on the polymerase active site structure and the balance of competing polymerase and exonuclease activities. A mismatch disfavors extension and favors the exonuclease.Fig 4-5 Superimposed structure of the Klenow fragment of DNA pol I with two different DNAs “Fingers” “Thumb” “Palm” red/orange helix: 3’ in red is elongating blue/cyan helix: 3’ in blue is getting edited Fig 4-6 E. -
Dna Replication in Archaea: Priming, Transferase, and Elongation Activities
DNA REPLICATION IN ARCHAEA: PRIMING, TRANSFERASE, AND ELONGATION ACTIVITIES by Zhongfeng Zuo Bachelor degree, Beijing Technology and Business University, 1999 Submitted to the Graduate Faculty of the Kenneth P. Dietrich School of Arts and Sciences in partial fulfillment of the requirements for the degree of Doctor of Philosophy University of Pittsburgh 2012 UNIVERSITY OF PITTSBURGH THE KENNETH P. DIETRICH SCHOOL OF ARTS AND SCIENCES This dissertation was presented by Zhongfeng Zuo It was defended on January 27, 2012 and approved by Stephen G. Weber, Professor, Department of Chemistry Billy Day, Professor, Department of Chemistry, Department of Pharmacy Renã A. S. Robinson, Assistant Professor, Department of Chemistry Dissertation Advisor: Michael A. Trakselis, Assistant Professor, Department of Chemistry ii DNA REPLICATION IN ARCHAEA: PRIMING, TRANSFERASE, AND ELONGATION ACTIVITIES Zhongfeng Zuo, Ph.D University of Pittsburgh, 2012 Copyright © by Zhongfeng Zuo 2012 iii DNA REPLICATION IN ARCHAEA: PRIMING, TRANSFERASE, AND ELONGATION ACTIVITIES Zhongfeng Zuo, Ph.D University of Pittsburgh, 2012 We have biochemically characterized the bacterial-like DnaG primase contained within the hyperthermophilic crenarchaeon Sulfolobus solfataricus (Sso ) and compared in vitro priming kinetics with those of the eukaryotic-like primase (PriS&L) also found in Sso . Sso DnaG exhibited metal- and temperature-dependent profiles consistent with priming at high temperatures. The distribution of primer products for Sso DnaG was discrete but highly similar to the distribution of primer products produced by the homologous Escherichia coli DnaG. The predominant primer length was 13 bases, although less abundant products of varying sizes are also present. Sso DnaG was found to bind DNA cooperatively as a dimer with a moderate dissociation constant. -
Recruitment of Terminal Protein to the Ends of Streptomyces Linear Plasmids and Chromosomes by a Novel Telomere-Binding Protein Essential for Linear DNA Replication
Downloaded from genesdev.cshlp.org on October 5, 2021 - Published by Cold Spring Harbor Laboratory Press Recruitment of terminal protein to the ends of Streptomyces linear plasmids and chromosomes by a novel telomere-binding protein essential for linear DNA replication Kai Bao1 and Stanley N. Cohen1,2,3 1Department of Genetics and 2Department of Medicine, Stanford University School of Medicine, Stanford, California 94305-5120, USA Bidirectional replication of Streptomyces linear plasmids and chromosomes from a central origin produces unpaired 3-leading-strand overhangs at the telomeres of replication intermediates. Filling in of these overhangs leaves a terminal protein attached covalently to the 5 DNA ends of mature replicons. We report here the essential role of a novel 80-kD DNA-binding protein (telomere-associated protein,Tap) in this process. Biochemical studies,yeast two-hybrid analysis,and immunopre cipitation/immunodepletion ,experiments indicate that Tap binds tightly to specific sequences in 3 overhangs and also interacts with Tpg bringing Tpg to telomere termini. Using DNA microarrays to analyze the chromosomes of tap mutant bacteria,we demonstrate that survivors of Tap ablation undergo telomere deletion,chromosome circularization,and amplification of subtelomeric DNA. Microarray-ba sed chromosome mapping at single-ORF resolution revealed common endpoints for independent deletions,identi fied amplified chromosomal ORFs adjacent to these endpoints,and quantified the copy number of these ORFs. Sequence analysis confirmed chromosome circularization and revealed the insertion of adventitious DNA between joined chromosome ends. Our results show that Tap is required for linear DNA replication in Streptomyces and suggest that it functions to recruit and position Tpg at the telomeres of replication intermediates. -
Chromosome Duplication in Saccharomyces Cerevisiae
| YEASTBOOK GENOME ORGANIZATION AND INTEGRITY Chromosome Duplication in Saccharomyces cerevisiae Stephen P. Bell*,1 and Karim Labib†,1 *Howard Hughes Medical Institute, Massachusetts Institute of Technology, Cambridge, Massachusetts 02139, and yMedical Research Council Protein Phosphorylation and Ubiquitylation Unit, Sir James Black Centre, School of Life Sciences, University of Dundee, DD1 5EH, United Kingdom ORCID ID: 0000-0002-2876-610X (S.P.B.) ABSTRACT The accurate and complete replication of genomic DNA is essential for all life. In eukaryotic cells, the assembly of the multi-enzyme replisomes that perform replication is divided into stages that occur at distinct phases of the cell cycle. Replicative DNA helicases are loaded around origins of DNA replication exclusively during G1 phase. The loaded helicases are then activated during S phase and associate with the replicative DNA polymerases and other accessory proteins. The function of the resulting replisomes is monitored by checkpoint proteins that protect arrested replisomes and inhibit new initiation when replication is inhibited. The replisome also coordinates nucleosome disassembly, assembly, and the establishment of sister chromatid cohesion. Finally, when two replisomes converge they are disassembled. Studies in Saccharomyces cerevisiae have led the way in our understanding of these processes. Here, we review our increasingly molecular understanding of these events and their regulation. KEYWORDS DNA replication; cell cycle; chromatin; chromosome duplication; genome stability; -
Changing Perspectives on the Role of Dnaa-ATP in Orisome Function and Timing Regulation
fmicb-10-02009 August 28, 2019 Time: 17:19 # 1 REVIEW published: 29 August 2019 doi: 10.3389/fmicb.2019.02009 Changing Perspectives on the Role of DnaA-ATP in Orisome Function and Timing Regulation Alan C. Leonard1*, Prassanna Rao2, Rohit P. Kadam1 and Julia E. Grimwade1 1 Laboratory of Microbial Genetics, Department of Biomedical and Chemical Engineering and Science, Florida Institute of Technology, Melbourne, FL, United States, 2 Department of Biochemistry, Vanderbilt University School of Medicine, Nashville, TN, United States Bacteria, like all cells, must precisely duplicate their genomes before they divide. Regulation of this critical process focuses on forming a pre-replicative nucleoprotein complex, termed the orisome. Orisomes perform two essential mechanical tasks that configure the unique chromosomal replication origin, oriC to start a new round of chromosome replication: (1) unwinding origin DNA and (2) assisting with loading of the replicative DNA helicase on exposed single strands. In Escherichia coli, a necessary orisome component is the ATP-bound form of the bacterial initiator protein, DnaA. DnaA- ATP differs from DnaA-ADP in its ability to oligomerize into helical filaments, and in its ability to access a subset of low affinity recognition sites in the E. coli replication origin. Edited by: The helical filaments have been proposed to play a role in both of the key mechanical Ludmila Chistoserdova, tasks, but recent studies raise new questions about whether they are mandatory for University of Washington, allADP United States orisome activity. It was recently shown that a version of E. coli oriC (oriC ), whose Reviewed by: multiple low affinity DnaA recognition sites bind DnaA-ATP and DnaA-ADP similarly, was Anders Løbner-Olesen, fully occupied and unwound by DnaA-ADP in vitro, and in vivo suppressed the lethality University of Copenhagen, Denmark of DnaA mutants defective in ATP binding and ATP-specific oligomerization. -
Cryptic Single-Stranded-DNA Binding Activities of the Phage P And
Proc. Natl. Acad. Sci. USA Vol. 94, pp. 1154–1159, February 1997 Biochemistry Cryptic single-stranded-DNA binding activities of the phage l P and Escherichia coli DnaC replication initiation proteins facilitate the transfer of E. coli DnaB helicase onto DNA (phage l DNA replicationyE. coli DNA replicationyregulation of DNA helicase action) BRIAN A. LEARN,SOO-JONG UM*, LI HUANG†, AND ROGER MCMACKEN‡ Department of Biochemistry, School of Hygiene and Public Health, Johns Hopkins University, 615 North Wolfe Street, Baltimore, MD 21205 Communicated by Thomas Kelly, Johns Hopkins University, Baltimore, MD, December 5, 1996 (received for review October 2, 1996) ABSTRACT The bacteriophage l P and Escherichia coli tight complex with the hexameric DnaB helicase (16) and the DnaC proteins are known to recruit the bacterial DnaB repli- helicase is recruited to the viral origin through interactions of the cative helicase to initiator complexes assembled at the phage and PzDnaB complex with the O-some (7, 11, 12). This second-stage bacterial origins, respectively. These specialized nucleoprotein nucleoprotein structure is seemingly unreactive until it is partially assemblies facilitate the transfer of one or more molecules of disassembled by the action of the E. coli DnaJ, DnaK, and GrpE DnaB helicase onto the chromosome; the transferred DnaB, in molecular chaperone system (8–10, 17). This disassembly reac- turn, promotes establishment of a processive replication fork tion stimulates DnaB helicase action by freeing DnaB from its apparatus. To learn more about the mechanism of the DnaB strong association with the P protein, an interaction which is transfer reaction, we investigated the interaction of replication known to suppress the ATPase and helicase activities of DnaB initiation proteins with single-stranded DNA (ssDNA). -
Cloning and Characterization of a Senescence Inducing and Class II Tumor Suppressor Gene in Ovarian Carcinoma at Chromosome Region 6Q27
Oncogene (2001) 20, 980 ± 988 ã 2001 Nature Publishing Group All rights reserved 0950 ± 9232/01 $15.00 www.nature.com/onc Cloning and characterization of a senescence inducing and class II tumor suppressor gene in ovarian carcinoma at chromosome region 6q27 Francesco Acquati1,8, Cristina Morelli2,8, Raaella Cinquetti1, Marco Giorgio Bianchi1, Davide Porrini1, Liliana Varesco3, Viviana Gismondi3, Romina Rocchetti4, Simona Talevi4, Laura Possati4, Chiara Magnanini2, Maria G Tibiletti5, Barbara Bernasconi5, Maria G Daidone6, Viji Shridhar7, David I Smith7, Massimo Negrini2, Giuseppe Barbanti-Brodano2 and Roberto Taramelli*,1 1Dipartimento di Biologia Strutturale e Funzionale, Universita' dell'Insubria, Varese, Italy; 2Dipartimento di Medicina Sperimentale e Diagnostica, Sezione di Microbiologia, UniversitaÁ di Ferrara, I-44100 Ferrara, Italy; 3Istituto Nazionale per la Ricerca sul Cancro Genova, Italy; 4Istituto di Scienze Biomediche, UniversitaÁ di Ancona, I-60131 Ancona, Italy; 5Laboratorio di Anatomia Patologica, Ospedale di Circolo, Varese, Italy; 6Dipartimento Oncologia Sperimentale, Istituto Nazionale Tumori, Milano, Italy; 7Division of Experimental Pathology, Department of Laboratory Medicine and Pathology, Mayo Clinic, Rochester, MN 55905, USA Cytogenetic, molecular and functional analysis has Introduction shown that chromosome region 6q27 harbors a senes- cence inducing gene and a tumor suppressor gene Abnormalities of the long arm of chromosome 6 are involved in several solid and hematologic malignancies. associated with several solid neoplasms including We have cloned at 6q27 and characterized the carcinomas of the ovary (Saito et al., 1992; Foulkes RNASE6PL gene which belongs to a family of et al., 1993; Cooke et al., 1996; Orphanos et al., 1995; cytoplasmic RNases highly conserved from plants, to Tibiletti et al., 1996), breast (Develee et al., 1991; man. -
DNA Or RNA Priming of Bacteriophage G4 DNA Synthesis By
Proc. Nati. Acad. Sci. USA Vol. 74, No. 7, pp. 2815-2819, July 1977 Biochemistry DNA or RNA priming of bacteriophage G4 DNA synthesis by Escherichia coli dnaG protein (ADP/DNA binding protein/bacteriophage ST-1 DNA/DNA replication) SUE WICKNER Laboratory of Molecular Biology, National Cancer Institute, National Institutes of Health, Bethesda, Maryland 20014 Communicated by Jerard Hurwitz, May 6, 1977 ABSTRACT Escherichia coli dnaG protein is involved in wild-type and thermolabile) were purified by procedures in the initiation of DNA synthesis dependent on G4 or ST-1 sin- refs. 13, 14, 15, 16, 5, and 17, respectively. Assay conditions and gle-stranded phage DNAs [Bouche, J.-P., Zechel, K. & Kornberg, definitions of units for DNA polymerase III are in ref. A. (1975)1. Biol. Chem. 250,5995-60011. The reaction occurs by 3; those the following mechanism: dnaG protein binds to specific sites for the other proteins are in the above references. DNA EF I on the DNA in a reaction requiring E. coli DNA binding protein. and DNA EF III were purified by procedures to be published An oligonucleotide is synthesized in a reaction involving dnaG elsewhere using the assay described in ref. 4. By native poly- protein, DNA binding protein, and DNA. With G4 DNA this acrylamide gel electrophoresis (18), the DNA binding protein reaction requires ADP, dTTP (or UTP), and dGTP (or GTP). was 80% pure and the dnaG protein from wild-type cells was Elongation of the oligonucleotide can be catalyzed by DNA 30% polymerase II or III in combination with dnaZ protein and pure. -
The Impact of the Integrated Stress Response on DNA Replication
The impact of the integrated stress response on DNA replication ____________________________________________________ Dissertation for the award of the degree "Doctor of Philosophy" (Ph.D.) Division of Mathematics and Natural Sciences of the Georg-August-Universität Göttingen within the doctoral program Molecular Biology of Cells of the Georg-August University School of Science (GAUSS) submitted by Josephine Ann Mun Yee Choo from Selangor, Malaysia Göttingen 2019 Thesis Committee 1. Prof. Dr. Matthias Dobbelstein, Institute of Molecular Oncology, University Medical Center Göttingen (UMG) 2. PD Dr. Halyna Shcherbata, Research Group – Gene Expression and Signaling, Max Planck Institute for Biophysical Chemistry (MPI-BPC) 3. Prof. Dr. Steven Johnsen, Clinic for General, Visceral and Pediatric Surgery, University Medical Center Göttingen (UMG) Members of the Examination Board 1st reviewer: Prof. Dr. Matthias Dobbelstein, Institute of Molecular Oncology, University Medical Center Göttingen (UMG) 2nd reviewer: PD Dr. Halyna Shcherbata, Research Group – Gene Expression and Signalling, Max Planck Institute for Biophysical Chemistry (MPI-BPC) External members of the Examination Board 1. Dr. Roland Dosch, Department of Developmental Biochemistry, University Medical Center Göttingen (UMG) 2. Prof. Dr. Heidi Hahn, Department of Human Genetics, University Medical Center Göttingen (UMG) 3. Prof. Dr. Dieter Kube, Department of Hematology and Oncology, University Medical Center Göttingen (UMG) 4. Dr. Nuno Raimundo, Department of Cellular Biochemistry, -
6.Start.Stop.07.Ppt [Read-Only]
Accessory factors summary 1. DNA polymerase can’t replicate a genome. Solution ATP? No single stranded template Helicase + The ss template is unstable SSB (RPA (euks)) - No primer Primase (+) No 3’-->5’ polymerase Replication fork Too slow and distributive SSB and sliding clamp - Sliding clamp can’t get on Clamp loader (γ/RFC) + Lagging strand contains RNA Pol I 5’-->3’ exo, RNAseH - Lagging strand is nicked DNA ligase + Helicase introduces + supercoils Topoisomerase II + and products tangled 2. DNA replication is fast and processive DNA polymerase holoenzyme 1 Maturation of Okazaki fragments Topoisomerases control chromosome topology Catenanes/knots Topos Relaxed/disentangled •Major therapeutic target - chemotherapeutics/antibacterials •Type II topos transport one DNA through another 2 Starting and stopping summary 1. DNA replication is controlled at the initiation step. 2. DNA replication starts at specific sites in E. coli and yeast. 3. In E. coli, DnaA recognizes OriC and promotes loading of the DnaB helicase by DnaC (helicase loader) 4. DnaA and DnaC reactions are coupled to ATP hydrolysis. 5. Bacterial chromosomes are circular, and termination occurs opposite OriC. 6. In E. coli, the helicase inhibitor protein, tus, binds 7 ter DNA sites to trap the replisome at the end. 7. Eukaryotic chromosomes are linear, and the chromosome ends cannot be replicated by the replisome. 8. Telomerase extends the leading strand at the end. 9. Telomerase is a ribonucleoprotein (RNP) with RNA (template) and reverse-transcriptase subunits. Isolating DNA sequences that mediate initiation 3 Different origin sequences in different organisms E. Coli (bacteria) OriC Yeast ARS (Autonomously Replicating Sequences) Metazoans ???? Initiation in prokaryotes and eukaryotes Bacteria Eukaryotes ORC + other proteins load MCM hexameric helicases MCM (helicase) + RPA (ssbp) Primase + DNA pol α PCNA:pol δ + RFC MCM (helicase) + RPA (ssbp) PCNA:pol δ + RFC (clamp loader) Primase + DNA pol α PCNA:pol δ + DNA ligase 4 Crystal structure of DnaA:ATP revealed mechanism of origin assembly 1.