Memory-Relevant Mushroom Body Output Synapses Are Cholinergic
Total Page:16
File Type:pdf, Size:1020Kb
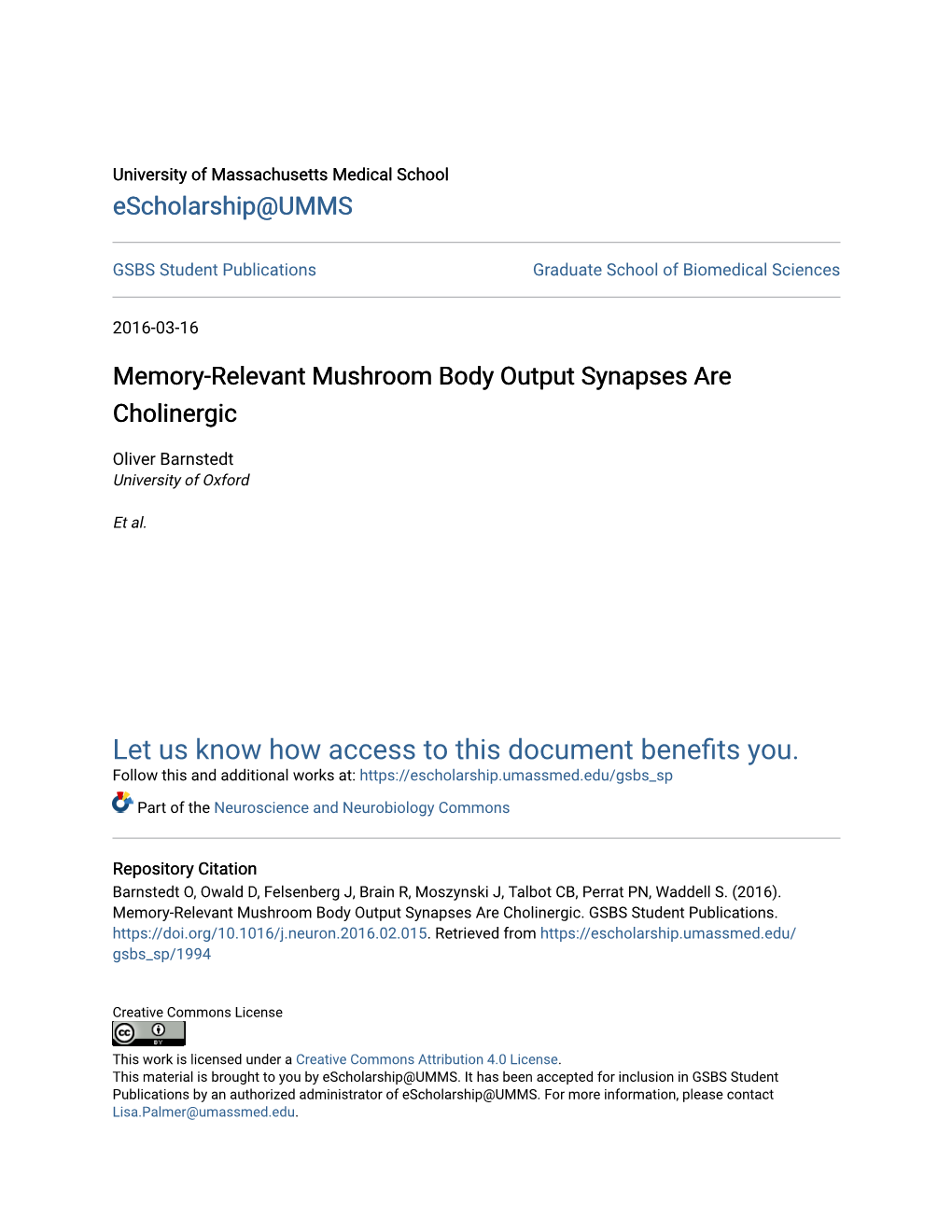
Load more
Recommended publications
-
Localized Olfactory Representation in Mushroom Bodies of Drosophila Larvae
Localized olfactory representation in mushroom bodies of Drosophila larvae Liria M. Masuda-Nakagawaa,1, Nanae¨ Gendreb, Cahir J. O’Kanec, and Reinhard F. Stockerb aInstitute of Molecular and Cellular Biosciences, University of Tokyo, Yayoi 1-1-1, Bunkyo-ku, Tokyo 113-0032, Japan; bDepartment of Biology, University of Fribourg, Chemin du Muse´e 10, CH-1700 Fribourg, Switzerland; and cDepartment of Genetics, University of Cambridge, Downing Street, Cambridge CB2 3EH, United Kingdom Edited by Obaid Siddiqi, Tata Institute for Fundamental Research, Bangalore, India, and approved May 4, 2009 (received for review January 7, 2009) Odor discrimination in higher brain centers is essential for behav- of spatial stereotypy of odor representations in PNs in the calyx ioral responses to odors. One such center is the mushroom body has not yet been functionally defined, and the complexity of the (MB) of insects, which is required for odor discrimination learning. adult calyx makes it difficult to visualize the representation of The calyx of the MB receives olfactory input from projection odor qualities in single identified cells. neurons (PNs) that are targets of olfactory sensory neurons (OSNs) Drosophila larvae, which can perceive a wide variety of odors in the antennal lobe (AL). In the calyx, olfactory information is (14) and perform odor discrimination learning (15, 16), have an transformed from broadly-tuned representations in PNs to sparse olfactory system with the same basic architecture as adults but representations in MB neurons (Kenyon cells). However, the extent numerically much simpler. It contains only 21 unique OSNs (17), of stereotypy in olfactory representations in the calyx is unknown. -
VIEW Open Access Structural Aspects of Plasticity in the Nervous System of Drosophila Atsushi Sugie1,2†, Giovanni Marchetti3† and Gaia Tavosanis3*
Sugie et al. Neural Development (2018) 13:14 https://doi.org/10.1186/s13064-018-0111-z REVIEW Open Access Structural aspects of plasticity in the nervous system of Drosophila Atsushi Sugie1,2†, Giovanni Marchetti3† and Gaia Tavosanis3* Abstract Neurons extend and retract dynamically their neurites during development to form complex morphologies and to reach out to their appropriate synaptic partners. Their capacity to undergo structural rearrangements is in part maintained during adult life when it supports the animal’s ability to adapt to a changing environment or to form lasting memories. Nonetheless, the signals triggering structural plasticity and the mechanisms that support it are not yet fully understood at the molecular level. Here, we focus on the nervous system of the fruit fly to ask to which extent activity modulates neuronal morphology and connectivity during development. Further, we summarize the evidence indicating that the adult nervous system of flies retains some capacity for structural plasticity at the synaptic or circuit level. For simplicity, we selected examples mostly derived from studies on the visual system and on the mushroom body, two regions of the fly brain with extensively studied neuroanatomy. Keywords: Structural plasticity, Drosophila, Photoreceptors, Synapse, Active zone, Mushroom body, Mushroom body calyx, Learning Background neuronal types, the feed-back derived from activity ap- The establishment of a functional neuronal circuit is a pears to be an important element to define which connec- dynamic process, including an extensive structural re- tions can be stabilized and which ones removed [3–5]. modeling and refinement of neuronal connections. Nonetheless, the cellular mechanisms initiated by activity Intrinsic differentiation programs and stereotypic mo- to drive structural remodeling during development and in lecular pathways contribute the groundwork of pattern- the course of adult life are not fully elucidated. -
A Review of Effects of Environment on Brain Size in Insects
insects Review A Review of Effects of Environment on Brain Size in Insects Thomas Carle Faculty of Biology, Kyushu University, Fukuoka 819-0395, Japan; [email protected] Simple Summary: What makes a big brain is fascinating since it is considered as a measure of intelligence. Above all, brain size is associated with body size. If species that have evolved with complex social behaviours possess relatively bigger brains than those deprived of such behaviours, this does not constitute the only factor affecting brain size. Other factors such as individual experience or surrounding environment also play roles in the size of the brain. In this review, I summarize the recent findings about the effects of environment on brain size in insects. I also discuss evidence about how the environment has an impact on sensory systems and influences brain size. Abstract: Brain size fascinates society as well as researchers since it is a measure often associated with intelligence and was used to define species with high “intellectual capabilities”. In general, brain size is correlated with body size. However, there are disparities in terms of relative brain size between species that may be explained by several factors such as the complexity of social behaviour, the ‘social brain hypothesis’, or learning and memory capabilities. These disparities are used to classify species according to an ‘encephalization quotient’. However, environment also has an important role on the development and evolution of brain size. In this review, I summarise the recent studies looking at the effects of environment on brain size in insects, and introduce the idea that the role of environment might be mediated through the relationship between olfaction and vision. -
Sprouting Interneurons in Mushroom Bodies of Adult Cricket Brains
THE JOURNAL OF COMPARATIVE NEUROLOGY 508:153–174 (2008) Sprouting Interneurons in Mushroom Bodies of Adult Cricket Brains ASHRAF MASHALY, MARGRET WINKLER, INA FRAMBACH, HERIBERT GRAS, AND FRIEDRICH-WILHELM SCHU¨ RMANN Johann-Friedrich-Blumenbach-Institut fu¨ r Zoologie und Anthropologie, Universita¨t Go¨ttingen, D-37073 Go¨ttingen, Germany ABSTRACT In crickets, neurogenesis persists throughout adulthood for certain local brain interneu- rons, the Kenyon cells in the mushroom bodies, which represent a prominent compartment for sensory integration, learning, and memory. Several classes of these neurons originate from a perikaryal layer, which includes a cluster of neuroblasts, surrounded by somata that provide the mushroom body’s columnar neuropil. We describe the form, distribution, and cytology of Kenyon cell groups in the process of generation and growth in comparison to developed parts of the mushroom bodies in adult crickets of the species Gryllus bimaculatus. A subset of growing Kenyon cells with sprouting processes has been distinguished from adjacent Kenyon cells by its prominent f-actin labelling. Growth cone-like elements are detected in the perikaryal layer and in their associated sprouting fiber bundles. Sprouting fibers distant from the perikarya contain ribosomes and rough endoplasmic reticulum not found in the dendritic processes of the calyx. A core of sprouting Kenyon cell processes is devoid of synapses and is not invaded by extrinsic neuronal elements. Measurements of fiber cross-sections and counts of synapses and organelles suggest a continuous gradient of growth and maturation leading from the core of added new processes out to the periphery of mature Kenyon cell fiber groups. Our results are discussed in the context of Kenyon cell classification, growth dynamics, axonal fiber maturation, and function. -
Genealogical Correspondence of Mushroom Bodies Across Invertebrate Phyla
Current Biology 25, 38–44, January 5, 2015 ª2015 Elsevier Ltd All rights reserved http://dx.doi.org/10.1016/j.cub.2014.10.049 Report Genealogical Correspondence of Mushroom Bodies across Invertebrate Phyla Gabriella H. Wolff1,* and Nicholas J. Strausfeld1,2 that are accorded the term ‘‘mushroom bodies’’ (insects) 1Department of Neuroscience, School of Mind, Brain, and and ‘‘hemiellipsoid bodies’’ (crustaceans). Four invariant Behavior, University of Arizona, Tucson, AZ 85721, USA characters define these centers’ ground pattern (Figure 1). 2Center for Insect Science, University of Arizona, Tucson, Clusters of basophilic globuli cells (Figures 1F and 1G) AZ 85721, USA giving rise to intrinsic fibers extending throughout the center provide approximately parallel arrangements (Figures 1H– 1K). Intrinsic fibers contribute to an orthogonal network with Summary arborizations of extrinsic (input/output) neurons (Figures 1H and 1J) and centrifugal cells that loop back to more distal Except in species that have undergone evolved loss, paired levels thereby forming feedback pathways (Figures 1D and lobed centers referred to as ‘‘mushroom bodies’’ occur 1E). The ground pattern is further defined by enriched expres- across invertebrate phyla [1–5]. Unresolved is the question sion of proteins essential for learning and memory functions in of whether these centers, which support learning and Drosophila (Figures 1F, 1G, and 2), namely, protein kinase memory in insects, correspond genealogically or whether A catalytic subunit alpha (DC0) [8]; Leonardo (Leo) [9], the their neuronal organization suggests convergent evolu- ortholog of mammalian 14-3-3z; and calcium/calmodulin- tion. Here, anatomical and immunohistological observations dependent protein kinase II (CaMKII) [10, 11]. -
Olfactory Coding in the Insect Brain: Molecular Receptive Ranges, Spatial and Temporal Coding
Olfactory coding in the insect brain: molecular receptive ranges, spatial and temporal coding C. Giovanni Galizia* & Paul Szyszka Departmellt ofNeurobiology, Urliversityo fKOlIStarlz, 78457 KOllstallz, Germany Key words: olfaction, receptor neurons, neural coding, antennal lobe, mushroom body, Kenyon cells, Drosophila melanogaster, Apis mellifera, combinatorial coding Abstract Odor information is coded in the insect brain in a sequence of steps, ranging from the receptor cells, via the neural network in the antennal lobe, to higher order brain centers, among which the mushroom bodies and the lateral horn are the most prominent. Across all of these processing steps, coding logic is combinatorial, in the sense that information is represented as patterns of activity across a population of neurons, rather than in individual neurons. Because different neurons are located in different places, such a coding logic is often termed spatial, and can be visualized with optical imaging techniques. We employ in vivo calcium imaging in order to record odor-evoked activity patterns in olfactory receptor neurons, different populations of local neurons in the antennal lobes, projection neurons linking antennal lobes to the mushroom bodies, and the intrinsic cells of the mushroom bodies themselves, the Kenyon cells. These studies confirmthe combinatorial nature of coding at all of these stages. However, the transmission of odor-evoked activity patterns from projection neuron dendrites via their axon terminals onto Kenyon cells is accompanied by a progressive sparsening of the population code. Activity patterns also show characteristic temporal properties. While a part of the temporal response properties reflect the physical sequence of odor filaments, another part is generated by local neuron networks. -
Clonal Analysis of the Mushroom Bodies 4067 Are Derived from the Same Nb Remains to Be Elucidated (Fig
Development 126, 4065-4076 (1999) 4065 Printed in Great Britain © The Company of Biologists Limited 1999 DEV8625 Development of the Drosophila mushroom bodies: sequential generation of three distinct types of neurons from a neuroblast Tzumin Lee, Arthur Lee and Liqun Luo* Department of Biological Sciences, Stanford University, Stanford, CA 94305, USA *Author for correspondence (e-mail: [email protected]) Accepted 9 July; published on WWW 23 August 1999 SUMMARY The mushroom bodies (MBs) are prominent structures in revealed how different neurons acquire their characteristic the Drosophila brain that are essential for olfactory axon projections. During the larval stage, axons of all MB learning and memory. Characterization of the development neurons bifurcate into both the dorsal and medial lobes. and projection patterns of individual MB neurons will be Shortly after puparium formation, larval MB neurons are important for elucidating their functions. Using mosaic selectively pruned according to birthdays. Degeneration of analysis with a repressible cell marker (Lee, T. and Luo, L. axon branches makes early-born (γ) neurons retain only (1999) Neuron 22, 451-461), we have positively marked the their main processes in the peduncle, which then project axons and dendrites of multicellular and single-cell into the adult γ lobe without bifurcation. In contrast, the mushroom body clones at specific developmental stages. basic axon projections of the later-born (α′/β′) larval Systematic clonal analysis demonstrates that a single neurons are preserved during metamorphosis. This study mushroom body neuroblast sequentially generates at least illustrates the cellular organization of mushroom bodies three types of morphologically distinct neurons. Neurons and the development of different MB neurons at the single projecting into the γ lobe of the adult MB are born first, cell level. -
Dop1r1, a Type 1 Dopaminergic Receptor Expressed in Mushroom Bodies, Modulates Drosophila Larval Locomotion
RESEARCH ARTICLE Dop1R1, a type 1 dopaminergic receptor expressed in Mushroom Bodies, modulates Drosophila larval locomotion 1,2 1,3 1,4 Bryon Silva , Sergio HidalgoID , Jorge M. CampusanoID * 1 Laboratorio NeurogeneÂtica de la Conducta, Departamento de BiologõÂa Celular y Molecular, Facultad de Ciencias BioloÂgicas, Pontificia Universidad CatoÂlica de Chile, Santiago, Chile, 2 Genes and Dynamics of Memory Systems, Brain Plasticity Unit, CNRS, ESPCI Paris, PSL Research University, Paris, France, a1111111111 3 School of Physiology, Pharmacology and Neuroscience, Faculty of Life Science, University of Bristol, a1111111111 Bristol, United Kingdom, 4 Centro Interdisciplinario de Neurociencias, Pontificia Universidad CatoÂlica de a1111111111 Chile, Santiago, Chile a1111111111 * [email protected] a1111111111 Abstract OPEN ACCESS As in vertebrates, dopaminergic neural systems are key regulators of motor programs in insects, including the fly Drosophila melanogaster. Dopaminergic systems innervate the Citation: Silva B, Hidalgo S, Campusano JM (2020) Dop1R1, a type 1 dopaminergic receptor Mushroom Bodies (MB), an important association area in the insect brain primarily associ- expressed in Mushroom Bodies, modulates ated to olfactory learning and memory, but that has been also implicated with the execution Drosophila larval locomotion. PLoS ONE 15(2): of motor programs. The main objectives of this work is to assess the idea that dopaminergic e0229671. https://doi.org/10.1371/journal. systems contribute to the execution of motor programs in Drosophila larvae, and then, to pone.0229671 evaluate the contribution of specific dopaminergic receptors expressed in MB to these pro- Editor: Efthimios M. C. Skoulakis, Biomedical grams. Our results show that animals bearing a mutation in the dopamine transporter show Sciences Research Center Alexander Fleming, GREECE reduced locomotion, while mutants for the dopaminergic biosynthetic enzymes or the dopa- mine receptor Dop1R1 exhibit increased locomotion. -
Sparse Odor Representation and Olfactory Learning
ARTICLES Sparse odor representation and olfactory learning Iori Ito1,4, Rose Chik-ying Ong1,2,4, Baranidharan Raman1,3 & Mark Stopfer1 Sensory systems create neural representations of environmental stimuli and these representations can be associated with other stimuli through learning. Are spike patterns the neural representations that get directly associated with reinforcement during conditioning? In the moth Manduca sexta, we found that odor presentations that support associative conditioning elicited only one or two spikes on the odor’s onset (and sometimes offset) in each of a small fraction of Kenyon cells. Using associative conditioning procedures that effectively induced learning and varying the timing of reinforcement relative to spiking in Kenyon cells, we found that odor-elicited spiking in these cells ended well before the reinforcement was delivered. Furthermore, increasing the temporal overlap between spiking in Kenyon cells and reinforcement presentation actually reduced the efficacy of learning. Thus, spikes in Kenyon cells do not constitute the odor representation that coincides with reinforcement, and Hebbian spike timing–dependent plasticity in Kenyon cells alone cannot underlie this learning. http://www.nature.com/natureneuroscience The sense of smell is very flexible. For animals, odors can take on synaptic transmission from Kenyon cells13–15 prevents insects from arbitrary meanings as warranted by the changing environment. Under- forming or retaining associative memories. In Drosophila,workwith standing how olfactory stimuli are represented in the brain is a mutants suffering from memory deficits found that proteins critical for prerequisite for studying how such representations become associated memory are concentrated in the mushroom bodies16. with other modalities. To understand how neural representations of odors become asso- Therelativelysimplestructureoftheinsectbrainmakesitusefulfor ciated with reinforcement stimuli, we first sought to characterize the studying the neural bases of sensory coding and associative learning. -
Odor Processing in the Bee: a Preliminary Study of the Role of Central Input to the Antennal Lobe
Odor Processing in the Bee: a Preliminary Study of the Role of Central Input to the Antennal Lobe. Christiane Linster David Marsan ESPeI, Laboratoire d'Electronique 10, Rue Vauquelin, 75005 Paris [email protected] Claudine Masson Michel Kerszberg Laboratoire de Neurobiologie Comparee Institut Pasteur des Invertebrees CNRS (URA 1284) INRNCNRS (URA 1190) Neurobiologie Moleculaire 91140 Bures sur Yvette, France 25, Rue du Dr. Roux [email protected] 75015 Paris, France Abstract Based on precise anatomical data of the bee's olfactory system, we propose an investigation of the possible mechanisms of modulation and control between the two levels of olfactory information processing: the antennallobe glomeruli and the mushroom bodies. We use simplified neurons, but realistic architecture. As a first conclusion, we postulate that the feature extraction performed by the antennallobe (glomeruli and interneurons) necessitates central input from the mushroom bodies for fine tuning. The central input thus facilitates the evolution from fuzzy olfactory images in the glomerular layer towards more focussed images upon odor presentation. 1. Introduction Honeybee foraging behavior is based on discrimination among complex odors which is the result of a memory process involving extraction and recall of "key-features" representative of the plant aroma (for a review see Masson et al. 1993). The study of the neural correlates of such mechanisms requires a determination of how the olfactory system successively analyses odors at each stage (namely: receptor cells, antennal lobe interneurons and glomeruli, mushroom bodies). Thus far, all experimental studies suggest the implication of both antennallobe and mushroom bodies in these processes. The signal transmitted by the receptor cells is essentially unstable and fluctuating. -
A Kinase-Dependent Feedforward Loop Affects CREBB Stability and Long
RESEARCH ARTICLE A kinase-dependent feedforward loop affects CREBB stability and long term memory formation Pei-Tseng Lee1, Guang Lin1, Wen-Wen Lin1, Fengqiu Diao2, Benjamin H White2, Hugo J Bellen1,3,4,5,6* 1Department of Molecular and Human Genetics, Baylor College of Medicine, Houston, United States; 2Laboratory of Molecular Biology, National Institute of Mental Health, National Institutes of Health, Bethesda, United States; 3Howard Hughes Medical Institute, Baylor College of Medicine, Houston, United States; 4Program in Developmental Biology, Baylor College of Medicine, Houston, United States; 5Department of Neuroscience, Baylor College of Medicine, Houston, United States; 6Jan and Dan Duncan Neurological Research Institute, Texas Children’s Hospital, Houston, United States Abstract In Drosophila, long-term memory (LTM) requires the cAMP-dependent transcription factor CREBB, expressed in the mushroom bodies (MB) and phosphorylated by PKA. To identify other kinases required for memory formation, we integrated Trojan exons encoding T2A-GAL4 into genes encoding putative kinases and selected for genes expressed in MB. These lines were screened for learning/memory deficits using UAS-RNAi knockdown based on an olfactory aversive conditioning assay. We identified a novel, conserved kinase, Meng-Po (MP, CG11221, SBK1 in human), the loss of which severely affects 3 hr memory and 24 hr LTM, but not learning. Remarkably, memory is lost upon removal of the MP protein in adult MB but restored upon its *For correspondence: reintroduction. Overexpression of MP in MB significantly increases LTM in wild-type flies showing [email protected] that MP is a limiting factor for LTM. We show that PKA phosphorylates MP and that both proteins synergize in a feedforward loop to control CREBB levels and LTM. -
Transformation of Odor Selectivity from Projection Neurons to Single Mushroom Body Neurons Mapped with Dual-Color Calcium Imaging
Transformation of odor selectivity from projection neurons to single mushroom body neurons mapped with dual-color calcium imaging Hao Lia,b, Yiming Lia,b, Zhengchang Leia,b, Kaiyu Wanga, and Aike Guoa,c,1 aInstitute of Neuroscience and State Key Laboratory of Neuroscience, Shanghai Institutes for Biological Sciences, Chinese Academy of Sciences, Shanghai 200031, China; bUniversity of Chinese Academy of Sciences, Shanghai 200031, China; and cState Key Laboratory of Brain and Cognitive Science, Institute of Biophysics, Chinese Academy of Sciences, Beijing 100101, China Edited* by Mu-ming Poo, University of California, Berkeley, CA, and approved June 4, 2013 (received for review April 1, 2013) Although the response properties of most neurons are, to a large neurons of the MB, the Kenyon cells (KCs), receive inputs from extent, determined by the presynaptic inputs that they receive, the axon terminals of the projection neurons (PNs). Neural cir- comprehensive functional characterization of the presynaptic in- cuitry of the MB calyx offers particular technical advantages for puts of a single neuron remains elusive. Toward this goal, we the comprehensive mapping of synaptic connectivity because introduce a dual-color calcium imaging approach that simulta- a single KC receives only a small number of PN inputs and the neously monitors the responses of a single postsynaptic neuron connections between PNs and KCs form characteristic structures together with its presynaptic axon terminal inputs in vivo. As that are within the resolving power of light microscopy (11, 12). a model system, we applied the strategy to the feed-forward con- Despite the relatively simple circuit structure, a major trans- formation of odor-evoked response patterns was observed be- nections from the projection neurons (PNs) to the Kenyon cells Drosophila tween PNs and KCs, in that single KCs are highly selective for (KCs) in the mushroom body of and functionally map- particular odors (13–15), whereas PNs are more broadly tuned ped essentially all PN inputs for some of the KCs.