S41598-021-87118-W.Pdf
Total Page:16
File Type:pdf, Size:1020Kb
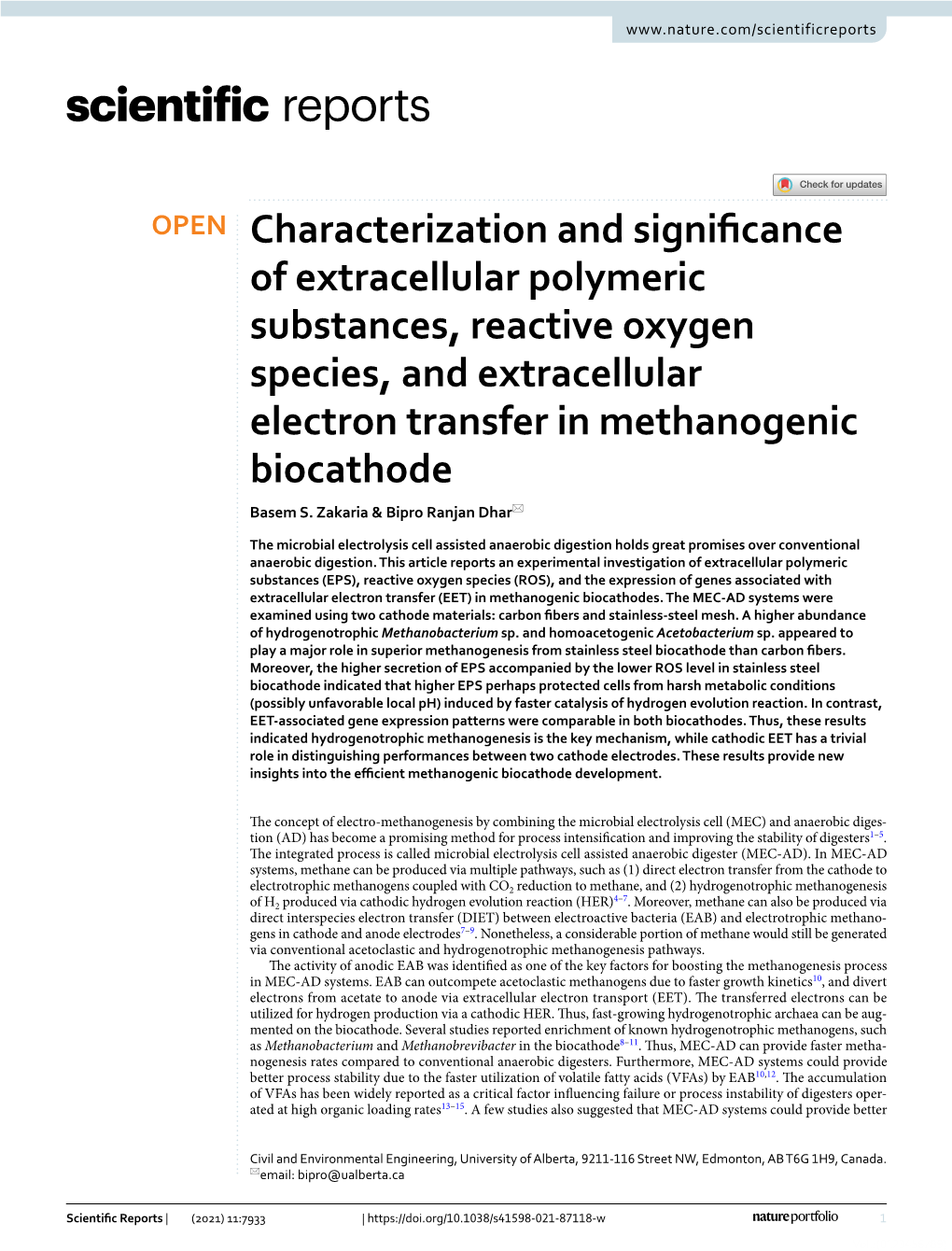
Load more
Recommended publications
-
Improving Microbial Electrosynthesis of Polyhydroxybutyrate (PHB) From
bioRxiv preprint doi: https://doi.org/10.1101/214577; this version posted November 7, 2017. The copyright holder for this preprint (which was not certified by peer review) is the author/funder. All rights reserved. No reuse allowed without permission. Improving microbial electrosynthesis of polyhydroxybutyrate (PHB) from CO2 by Rhodopseudomonas palustris TIE-1 using an immobilized iron complex modified cathode Karthikeyan Rengasamy, Tahina Onina Ranaivoarisoa, Rajesh Singh, Arpita Bose* Department of Biology, Washington University in Saint Louis, St. Louis, MO, 63130, USA. Abstract Microbial electrosynthesis (MES) is a promising bioelectrochemical approach to produce biochemicals. A previous study showed that Rhodopseudomonas palustris TIE-1 can directly use poised electrodes as electron donors for photoautotrophic growth at cathodic potentials that avoid electrolytic H2 production (photoelectroautotrophy). To make TIE-1 an effective biocatalyst for MES, we need to improve its electron uptake ability and growth under photoelectroautotrophic conditions. Because TIE-1 interacts with various forms of iron while using it as a source of electrons for photoautotrophy (photoferrotrophy), we tested the ability of iron-based redox mediators to enhance direct electron uptake. Our data show that soluble iron cannot act as a redox mediator for electron uptake by TIE-1 from a cathode poised at +100mV vs. Standard Hydrogen electrode. We then tested whether an immobilized iron-based redox mediator Prussian Blue (PB) can enhance electron uptake by TIE-1. Chronoamperometry indicates that cathodic current uptake by TIE-1 increased from 1.47 ± 0.04 to 5.6 ± 0.09 µA/cm2 (3.8 times) and the production of the bioplastic, polyhydroxybutyrate (PHB) improved from 13.5 ± 0.2 g/L to 18.8 ± 0.5 g/L (1.4 times) on electrodes coated with PB. -
Seasonal Energy Storage Potential Assessment of Wwtps with Power-To-Methane Technology
energies Article Seasonal Energy Storage Potential Assessment of WWTPs with Power-to-Methane Technology Zoltán Csed˝o 1,2, Botond Sinóros-Szabó 1 and Máté Zavarkó 1,2,* 1 Power-to-Gas Hungary Kft, 5000 Szolnok, Hungary; [email protected] (Z.C.); [email protected] (B.S.-S.) 2 Department of Management and Organization, Corvinus University of Budapest, 1093 Budapest, Hungary * Correspondence: [email protected] Received: 29 August 2020; Accepted: 18 September 2020; Published: 22 September 2020 Abstract: Power-to-methane technology (P2M) deployment at wastewater treatment plants (WWTPs) for seasonal energy storage might land on the agenda of decision-makers across EU countries, since large WWTPs produce a notable volume of biogas that could be injected into the natural gas grid with remarkable storage capacities. Because of the recent rapid increase of local photovoltaics (PV), it is essential to explore the role of WWTPs in energy storage and the conditions under which this potential can be realized. This study integrates a techno-economic assessment of P2M technology with commercial/investment attractiveness of seasonal energy storage at large WWTPs. Findings show that a standardized 1 MWel P2M technology would fit with most potential sites. This is in line with the current technology readiness level of P2M, but increasing electricity prices and limited financial resources of WWTPs would decrease the commercial attractiveness of P2M technology deployment. Based on a Hungarian case study, public funding, biomethane feed-in tariff and minimized or compensated surplus electricity sourcing costs are essential to realize the energy storage potential at WWTPs. Keywords: seasonal energy storage; power-to-methane; wastewater treatment plants; techno- economic assessment 1. -
Development of a Scalable Microbial Electrolysis Cell and Investigations of Exoelectrogenic Pure and Mixed Communities
The Pennsylvania State University The Graduate School College of Engineering DEVELOPMENT OF A SCALABLE MICROBIAL ELECTROLYSIS CELL AND INVESTIGATIONS OF EXOELECTROGENIC PURE AND MIXED COMMUNITIES A Dissertation in Environmental Engineering by Douglas F. Call © 2011 Douglas F. Call Submitted in Partial Fulfillment of the Requirements for the Degree of Doctor of Philosophy December 2011 The dissertation of Douglas F. Call was reviewed and approved* by the following: Bruce E. Logan Kappe Professor of Environmental Engineering Dissertation Advisor Chair of Committee John M. Regan Associate Professor of Environmental Engineering Brian A. Dempsey Professor of Environmental Engineering Ming Tien Professor of Biochemistry Peggy Johnson Professor of Civil Engineering Head of the Department of Civil and Environmental Engineering *Signatures are on file in the Graduate School ii ABSTRACT Bioelectrochemical systems (BESs) combine the fields of microbiology and electrochemistry for the production of electricity, gaseous fuels, or chemicals from biodegradable material. Advancing these systems towards large-scale applications requires improvements in both reactor designs and our understanding of the microbial ecology of exoelectrogenic biofilms. The work described in this dissertation addresses these areas through the development of a scalable microbial electrolysis cell (MEC), characterization of exoelectrogenic biofilms using the molecular technique fluorescent in- situ hybridization (FISH), and investigation into the current-producing and substrate- utilizing capabilities of the exoelectrogenic bacterium Geobacter sulfurreducens. Stainless Steel Brush Cathodes. MECs are a promising alternative method for producing hydrogen (H2) from biomass, yet they have been limited to the lab scale due in part to the inherent non-scalability and costs of electrodes. To address this problem, I investigated alternatives to the traditionally used platinized cathode. -
Microbial Electrolysis Cell Platform for Simultaneous Waste Biorefinery and Clean Electrofuels Generation: Current Situation, Ch
Progress in Energy and Combustion Science 63 (2017) 119À145 Contents lists available at ScienceDirect Progress in Energy and Combustion Science journal homepage: www.elsevier.com/locate/pecs Microbial electrolysis cell platform for simultaneous waste biorefinery and clean electrofuels generation: Current situation, challenges and future perspectives GuangyinTagedPD1XX ZhenD2XaX,b,*, XueqinD3XX LuD4XcX,**, GopalakrishnanD5XX KumarD6XdX, PD7XXeter BakonyiD8XeX, KaiqinD9XX XuD10XbX,**, YoucaiD1XX ZhaoD12XfX TagedPShanghai Key Lab for Urban Ecological Processes and Eco-Restoration, School of Ecological and Environmental Sciences, East China Normal University, Shanghai 200241, PR China b Center for Material Cycles and Waste Management Research, National Institute for Environmental Studies, 16-2 Onogawa, Tsukuba, Ibaraki 305-8506, Japan c Department of Civil and Environmental Engineering, Graduate School of Engineering, Tohoku University, Sendai, Miyagi 980-8579, Japan d Green Processing, Bioremediation and Alternative Energies Research Group, Faculty of Environment and Labour Safety, Ton Duc Thang University, Ho Chi Minh City, Vietnam e Research Institute on Bioengineering, Membrane Technology and Energetics, University of Pannonia, Egyetem ut 10, 8200 Veszprem, Hungary f The State Key Laboratory of Pollution Control and Resource Reuse, School of Environmental Science and Engineering, Tongji University, 200092, Shanghai, PR China ARTICLETAGEDP INFO ABSTRACTTAGEDP Article History: Microbial electrolysis cell (MEC) holds the flexible potentials -
Carbon Nanotubes Accelerate Acetoclastic Methanogenesis
Carbon nanotubes accelerate acetoclastic methanogenesis: From pure cultures to anaerobic soils Leilei Xiao, Shiling Zheng, Eric Lichtfouse, Min Luo, Yang Tan, Fanghua Liu To cite this version: Leilei Xiao, Shiling Zheng, Eric Lichtfouse, Min Luo, Yang Tan, et al.. Carbon nanotubes accelerate acetoclastic methanogenesis: From pure cultures to anaerobic soils. Soil Biology and Biochemistry, Elsevier, 2020, 150, 10.1016/j.soilbio.2020.107938. hal-02930808 HAL Id: hal-02930808 https://hal.archives-ouvertes.fr/hal-02930808 Submitted on 4 Sep 2020 HAL is a multi-disciplinary open access L’archive ouverte pluridisciplinaire HAL, est archive for the deposit and dissemination of sci- destinée au dépôt et à la diffusion de documents entific research documents, whether they are pub- scientifiques de niveau recherche, publiés ou non, lished or not. The documents may come from émanant des établissements d’enseignement et de teaching and research institutions in France or recherche français ou étrangers, des laboratoires abroad, or from public or private research centers. publics ou privés. Carbon nanotubes accelerate acetoclastic methanogenesis: From pure cultures to anaerobic soils Leilei Xiao a,e,f, Shiling Zheng a,f, Eric Lichtfouse c, Min Luo d, Yang Tan f, Fanghua Liu a,b,e,f,* a Key Laboratory of Coastal Biology and Biological Resources Utilization, Yantai Institute of Coastal Zone Research, Chinese Academy of Sciences, Yantai, 264003, PR China b Laboratory for Marine Biology and Biotechnology, Qingdao National Laboratory for Marine Science -
Biogas Upgrading / CO Reduction Using Renewable Hydrogen And
Biogas Upgrading / CO2 Reduction Using Renewable Hydrogen and Biocatalysts U.S. Department of Agriculture and Department of Energy Circular Carbon Economy Summit Denver, CO July 24-25, 2018 1 SoCalGas Largest U.S. natural gas distribution utility 140 years young Population 21 million 8,000 employees 1 Tcf/year 2 SoCalGas Transmission System Our Focus: Customer Needs and Emerging Trends Industry Themes Implications Solutions • Rising utility bills • Appliance energy efficiency • Develop technologies to meet air quality standards Affordability • Large percentage of customers on assistance • Utilize existing infrastructure and domestic supplies • Need to continue to provide affordable energy of natural gas • Acute public heath problem • Appliance emission standards • Renewable natural gas for transportation • Transportation is 80% of NOx Air Quality / NOx • Low Nox engines • Potential to replace diesel with clean fuels • Fuel cells • Demand for renewable gas • Renewable gas from biomass and excess wind & Greenhouse Gas • Wind and solar overgeneration (duck curve) solar power (power-to-gas) Emissions • Depressed power prices • Hydrogen for fuel and pipeline blending • Growing need for energy storage solutions • Electric and gas grid integration • Carbon up-cycling • Pipeline Monitoring Reliability and • Leak detection • Enhance pipeline safety Safety • Monitor and reduce methane emissions • Distributed energy applications • Robust power reliability 4 RD&D Objectives (PUC Code 740.1) 1. Environmental improvement 2. Public and employee safety 3. Conservation by efficient resource use or by reducing or shifting system load 4. Development of new resources and processes, particularly renewable resources and processes which further supply technologies 5. Improve operating efficiency and reliability or otherwise reduce operating costs 5 Research, Development and Demonstration (RD&D) End-Use Appliances Clean Transportation Emerging Technologies Low-carbon Resources . -
SU Agenda Template
University of Southampton Research Repository ePrints Soton Copyright © and Moral Rights for this thesis are retained by the author and/or other copyright owners. A copy can be downloaded for personal non-commercial research or study, without prior permission or charge. This thesis cannot be reproduced or quoted extensively from without first obtaining permission in writing from the copyright holder/s. The content must not be changed in any way or sold commercially in any format or medium without the formal permission of the copyright holders. When referring to this work, full bibliographic details including the author, title, awarding institution and date of the thesis must be given e.g. AUTHOR (year of submission) "Full thesis title", University of Southampton, name of the University School or Department, PhD Thesis, pagination http://eprints.soton.ac.uk UNIVERSITY OF SOUTHAMPTON FACULTY OF ENGINEERING AND THE ENVIRONMENT Mathematical Modelling and Simulation of Biofuel Cells by Mohamad Hussein Osman Thesis submitted for the degree of Doctor of Philosophy December 2013 UNIVERSITY OF SOUTHAMPTON ABSTRACT FACULTY OF ENGINEERING AND THE ENVIRONMENT MATHEMATICAL MODELLING AND SIMULATION OF BIOFUEL CELLS by Mohamad Hussein Osman Bio-fuel cells are driven by diverse and abundant bio-fuels and biological catalysts. The production/consumption cycle of bio-fuels is considered to be carbon neutral and, in princi- ple, more sustainable than that of conventional fuel cells. The cost benefits over traditional precious-metal catalysts, and the mild operating conditions represent further advantages. It is important that mathematical models are developed to reduce the burden on labo- ratory based testing and accelerate the development of practical systems. -
METHANOGENESIS Studies of the Process and the Micro-Organisms Involved in Methane Formation
METHANOGENESIS Studies of the process and the micro-organisms involved in methane formation Theo Hutten PROMOTOR: PROF.DR.IR. G.D. VOGELS CO-REFERENT: DR. С VAN DER DRIFT METHANOGENESIS STUDIES OF THE PROCESS AND THE MICRO-ORGANISMS INVOLVED IN METHANE FORMATION PROEFSCHRIFT TER VERKRIJGING VAN DE GRAAD VAN DOCTOR IN DE WISKUNDE EN NATUURWETENSCHAPPEN AAN DE KATHOLIEKE UNIVERSITEIT TE NIJMEGEN, OP GEZAG VAN DE RECTOR MAGNIFICUS PROF. DR. P.G.A.B. WIJDEVELD VOLGENS BESLUIT VAN HET COLLEGE VAN DEKANEN IN HET OPENBAAR TE VERDEDIGEN OP VRIJDAG 5 FEBRUARI 1982 DES NAMIDDAGS TE 2 UUR PRECIES door THEODORUS JACOBUS HENRI MARI HUTTEN geboren te Ootmarsum 1981 Druk: Snel druk Boulevard Enschede Gelukkig mag de eerste pagina in een proefschrift besteed worden aan al degenen die gedurende mijn studietijd en gedurende mijn promotie onderzoek veel voor mij.hebben betekend. Dank aan alle studenten die veel van het hierna beschreven onder zoek hebben verricht en die voor mij het dagelijkse noodzakelijke menselijke contact hebben betekend: Jan Keltjens, Peter van de Munckhof, Wim Robbers, Mieke Heuker of Hoek, Ed IJzerman, Gerard van Keulen, John Musters, Julie Kil, Retry van Kempen, Henk Kosse, Pieter Maas, Ben Peeters, Riek Bongaerts, Leon Gorris, Willem Pauli, Johan Heemskerk, Betty Bergman, Ton Breed en Rien de Goey. Mijn collega's - Mieke, Wim, John, Federico, Annemarie, Hans, Jan, Dick, Evert-Jan, Ger, Ton, Henk, Peter, Wolfgang, Claudius, Chris en Fried - wil ik bedanken voor hun niet aflatende hulp en vele genoeg lijke uren die we aan de koffie en met zeilen en sporten hebben door gebracht. De hulp en kennis die de Afdeling Preventieve en Sociale Tandheel kunde (Hans en Henk) en de Afdeling Antropogenetica (Frank) hebben geboden bij de isotachophorese, is zeer waardevol geweest. -
Methanogenesis in Oxygenated Soils Is a Substantial Fraction of Wetland Methane Emissions
Lawrence Berkeley National Laboratory Recent Work Title Methanogenesis in oxygenated soils is a substantial fraction of wetland methane emissions. Permalink https://escholarship.org/uc/item/6rm9360v Journal Nature communications, 8(1) ISSN 2041-1723 Authors Angle, Jordan C Morin, Timothy H Solden, Lindsey M et al. Publication Date 2017-11-16 DOI 10.1038/s41467-017-01753-4 Peer reviewed eScholarship.org Powered by the California Digital Library University of California ARTICLE DOI: 10.1038/s41467-017-01753-4 OPEN Methanogenesis in oxygenated soils is a substantial fraction of wetland methane emissions Jordan C. Angle1, Timothy H. Morin2,3, Lindsey M. Solden1, Adrienne B. Narrowe4, Garrett J. Smith 1, Mikayla A. Borton1,3, Camilo Rey-Sanchez2,3, Rebecca A. Daly1, Golnazalsdat Mirfenderesgi2, David W. Hoyt 5, William J. Riley6, Christopher S. Miller 4, Gil Bohrer 2,3 & Kelly C. Wrighton1,3 The current paradigm, widely incorporated in soil biogeochemical models, is that microbial 1234567890 methanogenesis can only occur in anoxic habitats. In contrast, here we show clear geo- chemical and biological evidence for methane production in well-oxygenated soils of a freshwater wetland. A comparison of oxic to anoxic soils reveal up to ten times greater methane production and nine times more methanogenesis activity in oxygenated soils. Metagenomic and metatranscriptomic sequencing recover the first near-complete genomes for a novel methanogen species, and show acetoclastic production from this organism was the dominant methanogenesis pathway in oxygenated soils. This organism, Candidatus Methanothrix paradoxum, is prevalent across methane emitting ecosystems, suggesting a global significance. Moreover, in this wetland, we estimate that up to 80% of methane fluxes could be attributed to methanogenesis in oxygenated soils. -
Microbial Methanogenesis in the Sulfate-Reducing Zone of Sediments in the Eckernförde Bay, SW Baltic Sea
Biogeosciences, 15, 137–157, 2018 https://doi.org/10.5194/bg-15-137-2018 © Author(s) 2018. This work is distributed under the Creative Commons Attribution 3.0 License. Microbial methanogenesis in the sulfate-reducing zone of sediments in the Eckernförde Bay, SW Baltic Sea Johanna Maltby1,a, Lea Steinle2,1, Carolin R. Löscher3,1, Hermann W. Bange1, Martin A. Fischer4, Mark Schmidt1, and Tina Treude5,6 1GEOMAR Helmholtz Centre for Ocean Research Kiel, Department of Marine Biogeochemistry, 24148 Kiel, Germany 2Department of Environmental Sciences, University of Basel, 4056 Basel, Switzerland 3Nordic Center for Earth Evolution, University of Southern Denmark, 5230 Odense, Denmark 4Institute of Microbiology, Christian-Albrecht-University Kiel, 24118 Kiel, Germany 5Department of Earth, Planetary, and Space Sciences, University of California Los Angeles (UCLA), Los Angeles, California 90095-1567, USA 6Department of Atmospheric and Oceanic Sciences, University of California Los Angeles (UCLA), Los Angeles, California 90095-1567, USA apresent address: Natural Sciences Department, Saint Joseph’s College, Standish, Maine 04084, USA Correspondence: Johanna Maltby ([email protected]) and Tina Treude ([email protected]) Received: 4 February 2017 – Discussion started: 7 March 2017 Revised: 30 October 2017 – Accepted: 8 November 2017 – Published: 10 January 2018 Abstract. Benthic microbial methanogenesis is a known way of methanogenesis in the presence or absence of sul- source of methane in marine systems. In most sediments, fate reducers, which after the addition of a noncompetitive the majority of methanogenesis is located below the sulfate- substrate was studied in four experimental setups: (1) un- reducing zone, as sulfate reducers outcompete methanogens altered sediment batch incubations (net methanogenesis), for the major substrates hydrogen and acetate. -
GCRP Tech Report 2012 Spormann-Logan
GCEP Technical Progress Report April 2013 Project: Capturing Electrical Current via Microbes to Produce Methane Alfred M. Spormann, Professor, Department of Chemical Engineering, and of Civil and Environmental Engineering, Stanford University; Bruce Logan, Professor, Department of Civil and Environmental Engineering, The Pennsylvania State University Svenja T. Lohner, postdoctoral researcher, Department of Civil and Environmental Engineering, Stanford University Michael Siegert, Douglas F. Call, postdoctoral researchers, Dept. of Civil and Environmental Engineering, The Pennsylvania State University Abstract The proposed microbial electromethanogenesis cell (MEMC) process will allow for the cost effec- tive production of methane from CO2 produced, for example, from gas/coal powered power plants or anaerobic digestion of bio-waste. The MEMC technology used will allow for production of CH4 with high efficiency relative to the electrical input. The technology is currently at the con- cept stage, with the scientific feasibility proven. However, the underlying science fundamentals such as the molecular and microbiological mechanisms of electron transfer from cathodes via in- termediates to cells and of the stability of the cathodic microbial communities are only insuffi- ciently understood. This lack of understanding presents the most significant bottleneck that needs to be solved before such technology can be deployed. The proposed research will advance both the science and the technology of this process, and the outcome should enable commerciali- zation of this technology. Background Recently, it has been discovered that CO2 can be directly converted to methane by microorganisms that harvest electrical energy from a cathode of a microbial electrolysis cell (Cheng et al. 2009). While the mechanism(s) behind “electromethanogenesis” are not fully understood, the experiment has been repeated by independent laboratories and is currently under development for industrial applications. -
Anaerobic Digestion Basics
CIS 1215 Anaerobic Digestion Basics Lide Chen and Howard Neibling pathogens and weed seeds associated with manure, Introduction improve manure nutrient availability to plants, and Dairy, potatoes, and sugar beets—all important sometimes generate new revenues. agricultural commodities in Idaho—also generate a huge amount of wastes. Two big waste-related What Is Anaerobic Digestion? challenges facing the dairy and food processing industries are emissions of odors and gases and Anaerobic digestion is a series of biological processes manure management. Gas emissions include that use a diverse population of bacteria to break down organic materials into biogas, primarily methane, and a greenhouse gases; ammonia (NH 3), a colorless gas with combination of solid and liquid effluents, the digestate a pungent smell; and hydrogen sulfide (H 2S), a colorless gas with the foul odor of rotten eggs. (figure 1). It occurs in the absence of free oxygen. Rising energy prices, more restrictive regulatory Organic materials are composed of organic compounds requirements, and increasing concern over greenhouse resulting from the remains or decomposition of previously gas emissions are causing many people in Idaho’s dairy living organisms such as plants and animals and their and food-processing industries to consider anaerobic waste products. Sources of organic material for anaerobic digestion (AD) of wastes from their operations. digestion include dairy manure, food processing waste, Anaerobic digestion technology is being viewed as a plant residues, and other organic wastes such as municipal way to address environmental concerns, generate wastewater, food waste, and fats, oils, and grease. renewable energy, cut bedding-material costs, reduce Other organic wastes Heat Electricity H2S removal Animal waste s ga io b ne ha et Electricity Biogas M generator Pu re m et ha Cow bedding Digester reactor ne CO and 2 Natural gas pipeline impurities High-quality, low-odor fertilizer Figure 1.