A Highly Conserved Region Essential for NMD in the Upf2 N-Terminal Domain
Total Page:16
File Type:pdf, Size:1020Kb
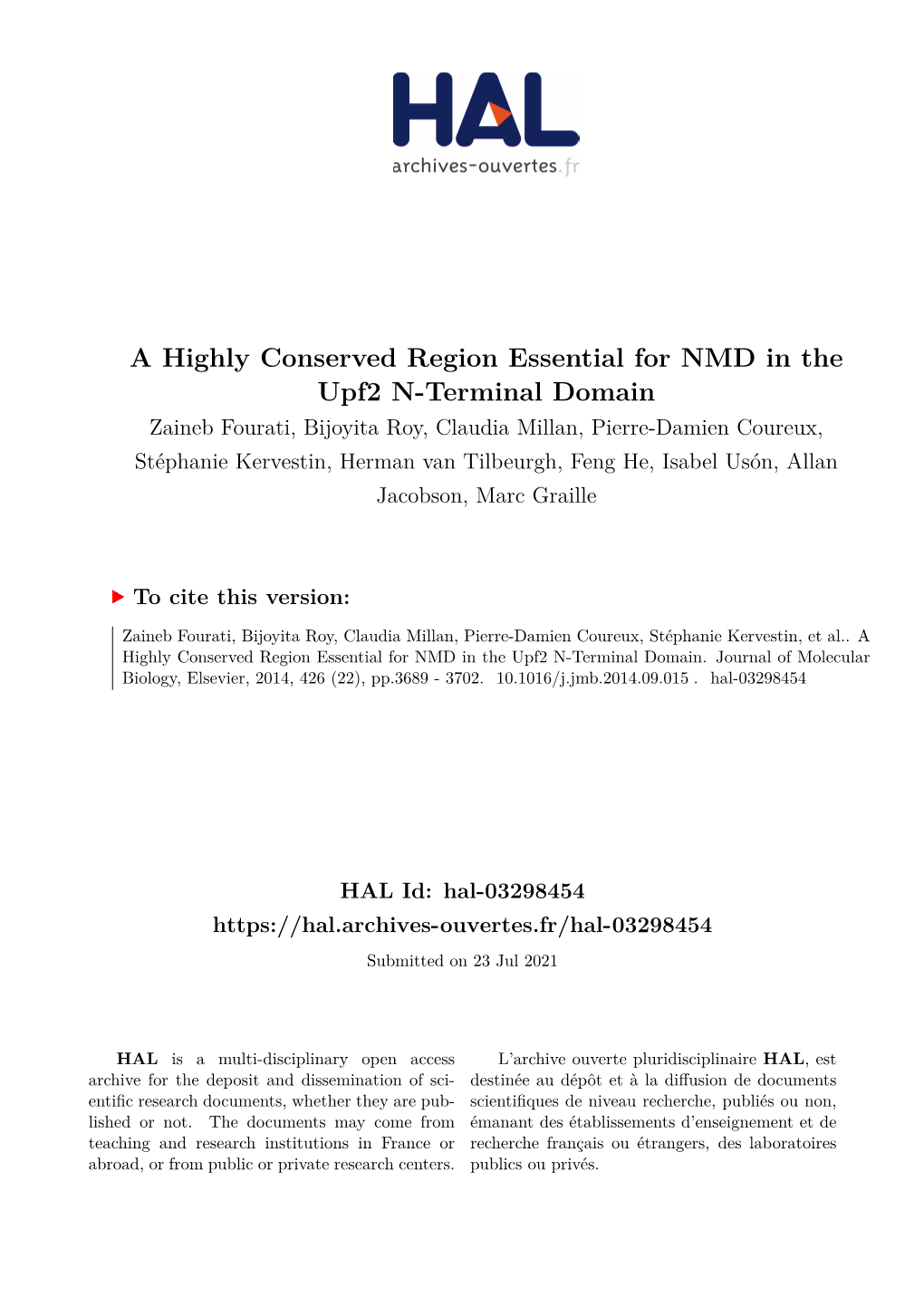
Load more
Recommended publications
-
Crystal Structure of the UPF2-Interacting Domain of Nonsense-Mediated Mrna Decay Factor UPF1
JOBNAME: RNA 12#10 2006 PAGE: 1 OUTPUT: Friday September 8 11:24:46 2006 csh/RNA/122854/rna1776 Downloaded from rnajournal.cshlp.org on September 28, 2021 - Published by Cold Spring Harbor Laboratory Press Crystal structure of the UPF2-interacting domain of nonsense-mediated mRNA decay factor UPF1 JAN KADLEC, DELPHINE GUILLIGAY, RAIMOND B. RAVELLI, and STEPHEN CUSACK European Molecular Biology Laboratory, Grenoble Outstation, BP 181, 38042 Grenoble Cedex 9, France ABSTRACT UPF1 is an essential eukaryotic RNA helicase that plays a key role in various mRNA degradation pathways, notably nonsense- mediated mRNA decay (NMD). In combination with UPF2 and UPF3, it forms part of the surveillance complex that detects mRNAs containing premature stop codons and triggers their degradation in all organisms studied from yeast to human. We describe the 3 A˚ resolution crystal structure of the highly conserved cysteine–histidine-rich domain of human UPF1 and show that it is a unique combination of three zinc-binding motifs arranged into two tandem modules related to the RING-box and U-box domains of ubiquitin ligases. This UPF1 domain interacts with UPF2, and we identified by mutational analysis residues in two distinct conserved surface regions of UPF1 that mediate this interaction. UPF1 residues we identify as important for the interaction with UPF2 are not conserved in UPF1 homologs from certain unicellular parasites that also appear to lack UPF2 in their genomes. Keywords: nonsense-mediated mRNA decay; NMD; surveillance complex; UPF1; X-ray crystallography INTRODUCTION from the mRNA. If, however, translation terminates at a PTC upstream of an EJC, UPF2 associated with a down- Nonsense-mediated mRNA decay (NMD) is an mRNA stream EJC can be bound by UPF1 that is recruited to the degradation pathway that detects and eliminates aberrant terminating ribosome within the so-called SURF complex, coding transcripts containing premature termination codons which also includes the translation release factors eRF1 and (PTC) originating from nonsense or frameshift mutations. -
Germ Granule-Mediated RNA Regulation in Male Germ Cells
REPRODUCTIONREVIEW Germ granule-mediated RNA regulation in male germ cells Tiina Lehtiniemi and Noora Kotaja Institute of Biomedicine, University of Turku, Turku, Finland Correspondence should be addressed to N Kotaja; Email: [email protected] Abstract Germ cells have exceptionally diverse transcriptomes. Furthermore, the progress of spermatogenesis is accompanied by dramatic changes in gene expression patterns, the most drastic of them being near-to-complete transcriptional silencing during the final steps of differentiation. Therefore, accurate RNA regulatory mechanisms are critical for normal spermatogenesis. Cytoplasmic germ cell-specific ribonucleoprotein (RNP) granules, known as germ granules, participate in posttranscriptional regulation in developing male germ cells. Particularly, germ granules provide platforms for the PIWI-interacting RNA (piRNA) pathway and appear to be involved both in piRNA biogenesis and piRNA-targeted RNA degradation. Recently, other RNA regulatory mechanisms, such as the nonsense-mediated mRNA decay pathway have also been associated to germ granules providing new exciting insights into the function of germ granules. In this review article, we will summarize our current knowledge on the role of germ granules in the control of mammalian male germ cell’s transcriptome and in the maintenance of fertility. Reproduction (2018) 155 R77–R91 Introduction then rapidly undergo the second meiotic division (meiosis II) resulting in haploid spermatids. The final Spermatogenesis is a highly specialized process that phase of spermatogenesis is haploid differentiation aims to transmit correct paternal genetic and epigenetic (spermiogenesis), which includes dramatic information to the next generation. At the embryonic morphological changes through which spermatozoa stage, the mammalian germ cell lineage is specified reach their dynamic sleek shape (Fig. -
Dissertation
Regulation of gene silencing: From microRNA biogenesis to post-translational modifications of TNRC6 complexes DISSERTATION zur Erlangung des DOKTORGRADES DER NATURWISSENSCHAFTEN (Dr. rer. nat.) der Fakultät Biologie und Vorklinische Medizin der Universität Regensburg vorgelegt von Johannes Danner aus Eggenfelden im Jahr 2017 Das Promotionsgesuch wurde eingereicht am: 12.09.2017 Die Arbeit wurde angeleitet von: Prof. Dr. Gunter Meister Johannes Danner Summary ‘From microRNA biogenesis to post-translational modifications of TNRC6 complexes’ summarizes the two main projects, beginning with the influence of specific RNA binding proteins on miRNA biogenesis processes. The fate of the mature miRNA is determined by the incorporation into Argonaute proteins followed by a complex formation with TNRC6 proteins as core molecules of gene silencing complexes. miRNAs are transcribed as stem-loop structured primary transcripts (pri-miRNA) by Pol II. The further nuclear processing is carried out by the microprocessor complex containing the RNase III enzyme Drosha, which cleaves the pri-miRNA to precursor-miRNA (pre-miRNA). After Exportin-5 mediated transport of the pre-miRNA to the cytoplasm, the RNase III enzyme Dicer cleaves off the terminal loop resulting in a 21-24 nt long double-stranded RNA. One of the strands is incorporated in the RNA-induced silencing complex (RISC), where it directly interacts with a member of the Argonaute protein family. The miRNA guides the mature RISC complex to partially complementary target sites on mRNAs leading to gene silencing. During this process TNRC6 proteins interact with Argonaute and recruit additional factors to mediate translational repression and target mRNA destabilization through deadenylation and decapping leading to mRNA decay. -
Understanding Regulation of Mrna by RNA Binding Proteins Alexander
Understanding Regulation of mRNA by RNA Binding Proteins MA SSACHUSETTS INSTITUTE by OF TECHNOLOGY Alexander De Jong Robertson B.S., Stanford University (2008) LIBRARIES Submitted to the Graduate Program in Computational and Systems Biology in partial fulfillment of the requirements for the degree of Doctor of Philosophy in Computational and Systems Biology at the MASSACHUSETTS INSTITUTE OF TECHNOLOGY February 2014 o Massachusetts Institute of Technology 2014. All rights reserved. A A u th o r .... v ..... ... ................................................ Graduate Program in Computational and Systems Biology December 19th, 2013 C ertified by .............................................. Christopher B. Burge Professor Thesis Supervisor A ccepted by ........ ..... ............................. Christopher B. Burge Computational and Systems Biology Ph.D. Program Director 2 Understanding Regulation of mRNA by RNA Binding Proteins by Alexander De Jong Robertson Submitted to the Graduate Program in Computational and Systems Biology on December 19th, 2013, in partial fulfillment of the requirements for the degree of Doctor of Philosophy in Computational and Systems Biology Abstract Posttranscriptional regulation of mRNA by RNA-binding proteins plays key roles in regulating the transcriptome over the course of development, between tissues and in disease states. The specific interactions between mRNA and protein are controlled by the proteins' inherent affinities for different RNA sequences as well as other fea- tures such as translation and RNA structure which affect the accessibility of mRNA. The stabilities of mRNA transcripts are regulated by nonsense-mediated mRNA de- cay (NMD), a quality control degradation pathway. In this thesis, I present a novel method for high throughput characterization of the binding affinities of proteins for mRNA sequences and an integrative analysis of NMD using deep sequencing data. -
Description: Uniprot: F4IUX6 Source: Mouse Mol.Wt.: 134 Kda UPF2
#ZW049348 UPF2 Antibody for Plant Order 021-34695924 [email protected] Support 400-6123-828 50ul [email protected] 100 uL Web www.abmart.cn Description: Recruited by UPF3 associated with the EJC core at the cytoplasmic side of the nuclear envelope and the subsequent formation of an UPF1-UPF2-UPF3 surveillance complex (including UPF1 bound to release factors at the stalled ribosome) is believed to activate NMD. In cooperation with UPF3 stimulates both ATPase and RNA helicase activities of UPF1. Binds spliced mRNA (By similarity). Involved in nonsense-mediated decay (NMD) of mRNAs containing premature stop codons by associating with the nuclear exon junction complex (EJC). Required for plant development and adaptation to environmental stresses, including plant defense and response to wounding. Uniprot: F4IUX6 Alternative Names: Regulator of nonsense transcripts UPF2; Nonsense mRNA reducing factor UPF2; Up-frameshift suppressor 2 homolog; At2g39260; Reactivity: Arabidopsis thaliana Predicted reactivity: Solanum tuberosum; Capsicum annuum; Arabidopsis thaliana; Oryza sativa; Malus domestica; Prunus persica; Rosa chinensis; Gossypium mustelinum; Source: Mouse Mol.Wt.: 134 kDa Storage Condition: Store at -20 °C. Stable for 12 months from date of receipt. Application: WB 1:500-1:2000, IHC 1:50-1:200, IP: 1:50-1:200 Figure 2. UPF1, UPF2, and UPF3 Decay during an Early Phase of PstDC3000 Infection. (A) Dynamics of UPF1, UPF2, and UPF3 proteins up to 30 hpi (top) and 50 mpi (bottom). Immunoblot analyses (left) were performed for leaf samples taken from wild-type Col-0 plants that had been infected with Pseudomonas and collected at the indicated time points using an anti-UPF1 monoclonal antibody (a-UPF1), a-UPF2, ora-UPF3. -
Identification and Characterization Genes That Are Required . Or the Accelerated Degradauon of Mrnas Containing a Premature Translational Termination Codon
Downloaded from genesdev.cshlp.org on October 7, 2021 - Published by Cold Spring Harbor Laboratory Press Identification and characterization genes that are required . or the accelerated degradauon of mRNAs containing a premature translational termination codon Ying Cui, 1 Kevin W. Hagan, 1 Shuang Zhang, 2 and Stuart W. Peltz 1-3 ~Department of Molecular Genetics and Microbiology, Robert Wood Johnson Medical School, University of Medicine and Dentistry of New Jersey and 2the Program in Microbiology and Molecular Genetics, Rutgers University, Piscataway, New Jersey 08854 USA In both prokaryotes and eukaryotes nonsense mutations in a gene can enhance the decay rate of the mRNA transcribed from that gene, a phenomenon described as nonsense-mediated mRNA decay. In yeast, the products of the UPF1 and UPF3 genes are required for this decay pathway, and in this report we focus on the identification and characterization of additional factors required for rapid decay of nonsense-containing mRNAs. We present evidence that the product of the UPF2 gene is a new factor involved in this decay pathway. Mutation of the UPF2 gene or deletion of it from the chromosome resulted in stabilization of nonsense-containing mRNAs, whereas the decay of wild-type transcripts was not affected. The UPF2 gene was isolated, and its transcript was characterized. Our results demonstrate that the UPF2 gene encodes a putative 126.7-kD protein with an acidic region at its carboxyl terminus (-D-E)n found in many nucleolar and transcriptional activator proteins. The UPF2 transcript is 3600 nucleotides in length and contains an intron near its 5' end. -
Genomic Copy Number Variation Association Study in Caucasian Patients with Nonsyndromic Cryptorchidism Yanping Wang1, Jin Li3, Thomas F
Wang et al. BMC Urology (2016) 16:62 DOI 10.1186/s12894-016-0180-4 RESEARCH ARTICLE Open Access Genomic copy number variation association study in Caucasian patients with nonsyndromic cryptorchidism Yanping Wang1, Jin Li3, Thomas F. Kolon4, Alicia Olivant Fisher1, T. Ernesto Figueroa2, Ahmad H. BaniHani2, Jennifer A. Hagerty2, Ricardo Gonzalez2,9, Paul H. Noh2,10, Rosetta M. Chiavacci3, Kisha R. Harden3, Debra J. Abrams3, Deborah Stabley1, Cecilia E. Kim3, Katia Sol-Church1, Hakon Hakonarson3,5,6, Marcella Devoto5,6,7,8 and Julia Spencer Barthold1,2* Abstract Background: Copy number variation (CNV) is a potential contributing factor to many genetic diseases. Here we investigated the potential association of CNV with nonsyndromic cryptorchidism, the most common male congenital genitourinary defect, in a Caucasian population. Methods: Genome wide genotyping were performed in 559 cases and 1772 controls (Group 1) using Illumina HumanHap550 v1, HumanHap550 v3 or Human610-Quad platforms and in 353 cases and 1149 controls (Group 2) using the Illumina Human OmniExpress 12v1 or Human OmniExpress 12v1-1. Signal intensity data including log R ratio (LRR) and B allele frequency (BAF) for each single nucleotide polymorphism (SNP) were used for CNV detection using PennCNV software. After sample quality control, gene- and CNV-based association tests were performed using cleaned data from Group 1 (493 cases and 1586 controls) and Group 2 (307 cases and 1102 controls) using ParseCNV software. Meta-analysis was performed using gene-based test results as input to identify significant genes, and CNVs in or around significant genes were identified in CNV-based association test results. -
Abstracts [PDF]
Beyond the Identification of Transcribed Sequences 2002 Workshop Beyond the Identification of Transcribed Sequences: Functional, Evolutionary and Expression Analysis 12th International Workshop October 25-28, 2002 Washington, DC Sponsored by the U.S. Department of Energy Home * List of Abstracts * Speakers Meeting Objective Program Planning Committee: The 12th workshop in this series was held in Tom Freeman, MRC Human Genome Washington D.C., Friday evening, October 25 through Programme, Hinxton, UK Monday afternoon, October 28, 2002. Interested Katheleen Gardiner, Eleanor Roosevelt Institute, investigators actively engaged in any aspect of the Denver, CO, USA functional, expression or evolutionary analysis of Bernhard Korn, German Cancer Research Center, transcribed sequences were invited to send an abstract. Heidelberg, Germany Blair Hedges, Penn State University, University Topics discussed include but are not limited to: Park, PA, USA mammalian gene and genome organization as Sherman Weissman, Yale University, New determined from the construction of transcriptional Haven, CT, USA maps and genomic sequence analysis; expression Thomas Werner, Institute for Saeugertiergenetik, analysis of novel mammalian genes; analysis of Oberschleissheim, Germany genomic sequence, including gene and regulatory sequence prediction and verification, and annotation for For Questions or Additional Information, public databases; expression and mutation analysis, and contact: comparative mapping and genomic sequence analysis in model organisms (e.g. yeast, -
Proteasome Inhibitors and Knockdown of SMG1 Cause Accumulation of Upf1 and Upf2 in Human Cells
222 INTERNATIONAL JOURNAL OF ONCOLOGY 44: 222-228, 2014 Proteasome inhibitors and knockdown of SMG1 cause accumulation of Upf1 and Upf2 in human cells XIA ZHAO1,2, ATSUSHI NOGAWA3, TSUKASA MATSUNAGA3, TSUTOMU TAKEGAMI2, HIDEAKI NAKAGAWA2 and YASUHITO ISHIGAKI2 1Institute of Pathology, Tongji Hospital, Tongji Medical College, Huazhong University of Science and Technology, Wuhan 430030, P.R. China; 2Medical Research Institute, Kanazawa Medical University, Kahoku 920-0293; 3Laboratory of Human Molecular Genetics, School of Pharmaceutical Sciences, Kanazawa University, Kakuma, Kanazawa 920-1192, Japan Received July 27, 2013; Accepted September 23, 2013 DOI: 10.3892/ijo.2013.2149 Abstract. The ubiquitin-proteasome system (UPS) is one biological processes such as proteins turnover, cell cycle of the most promising anticancer drug targets of the century. control, antigen processing, signal transduction, protein However, the involved molecular mechanisms are still unclear. quality control, cell differentiation and apoptosis (1,2). It has The nonsense-mediated mRNA decay (NMD) pathway been found that tumor cells are more sensitive to proteasome is a highly conserved pathway which degrades nonsense inhibitors than normal cells. Today, the proteasome is one of mutation‑containing mRNA selectively and efficiently. In this the most promising targets of anticancer drug, but the involved pathway, the SMG1-Upf1-eRF (SURF) complex binds to Upf2 molecular mechanisms are still unclear. on the exon junction complex and finally causes degradation Gene expression is regulated by various mechanisms in of nonsense-containing mRNA. To reveal the relationship which RNA decay pathway is one of the most important regu- between the UPS and NMD pathways, we analyzed the effects lators. -
Supplementary Figures Compartmentalized Nonsense-Mediated Mrna Decay Regulates Synaptic Plasticity and Cognitive Function Via Gl
Supplementary Figures Compartmentalized nonsense-mediated mRNA decay regulates synaptic plasticity and cognitive function via GluR1 signaling Michael Notaras1, Megan Allen1, Francesco Longo2, Nicole Volk1, Miklos Toth3, Noo Li Jeon4, Eric Klann2, Dilek Colak1,5, * 1 Center for Neurogenetics, Feil Family Brain and Mind Research Institute, Weill Cornell Medical College, Cornell University, New York City, New York, USA. 2 Center for Neural Science, New York University, New York City, New York, USA. 3 Department of Pharmacology, Weill Cornell Medical College, Cornell University, New York City, New York, USA. 4 School of Mechanical and Aerospace Engineering, Seoul National University, Seoul, South Korea. 5 Gale & Ira Drukier Institute for Children’s Health, Weill Cornell Medical College, Cornell University, New York City, New York, USA. *Correspondence: [email protected] Fig. S1 | Knockdown of UPF2 in mouse hippocampal neurons by UPF2- shRNA:GFP lentivirus. To disrupt NMD, we targeted the UPF2 mRNA using UPF2-shRNA:GFP lentivirus. Canonical NMD is completed by the interaction of UPF1, UPF2 and UPF3 proteins. While UPF1 function is not restricted to the NMD pathway [1], UPF2 has been successfully used to disrupt NMD in several studies [2-4]. We infected E16 hippocampal neurons at DIV7 with control- or UPF2-shRNA virus. To assess the knockdown of UPF2, we fixed the cells at DIV14 and performed immunohistochemistry for UPF2. UPF2-shRNA application resulted in a robust depletion of UPF2 within infected neurons and their dendrites, while UPF2 expression remained intact in scrambled control-shRNA cultures and non-infected neurons in UPF2-shRNA cultures (UPF2+, GFP- cells in UPF2-shRNA panel). -
UPF3A and UPF3B Are Redundant and Modular Activators of Nonsense-Mediated Mrna Decay in Human Cells
bioRxiv preprint doi: https://doi.org/10.1101/2021.07.07.451444; this version posted July 13, 2021. The copyright holder for this preprint (which was not certified by peer review) is the author/funder, who has granted bioRxiv a license to display the preprint in perpetuity. It is made available under aCC-BY-NC 4.0 International license. 1 UPF3A and UPF3B are redundant and modular activators of 2 nonsense-mediated mRNA decay in human cells 3 4 Damaris Wallmeroth1,2, Volker Boehm1,2, Jan-Wilm Lackmann3, Janine Altmüller4,5, Christoph 5 Dieterich6,7, Niels H. Gehring1,2 6 7 1 Institute for Genetics, University of Cologne, 50674 Cologne, Germany 8 2 Center for Molecular Medicine Cologne (CMMC), University of Cologne, 50937 Cologne, 9 Germany 10 3 CECAD Research Center, University of Cologne, Joseph-Stelzmann-Str. 26, 50931 11 Cologne, Germany 12 4 Cologne Center for Genomics (CCG), University of Cologne, 50931 Cologne, Germany 13 5 Present address: Berlin Institute of Health at Charité – Universitätsmedizin Berlin, Core 14 Facility Genomics, Charitéplatz 1, 10117 Berlin, Germany and Max Delbrück Center for 15 Molecular Medicine in the Helmholtz Association (MDC), Berlin, Germany 16 6 Section of Bioinformatics and Systems Cardiology, Department of Internal Medicine III and 17 Klaus Tschira Institute for Integrative Computational Cardiology, Heidelberg University 18 Hospital, 69120 Heidelberg, Germany 19 7 DZHK (German Centre for Cardiovascular Research), Partner site Heidelberg/Mannheim, 20 69120 Heidelberg, Germany 21 22 *Correspondence: [email protected] (V.B.), [email protected] (N.H.G.) 23 24 Keywords 25 Nonsense-mediated mRNA decay, gene paralogs, mRNA turnover, UPF3 26 1 bioRxiv preprint doi: https://doi.org/10.1101/2021.07.07.451444; this version posted July 13, 2021. -
The RNA Surveillance Proteins UPF1, UPF2 and SMG6 Affect HIV-1 Reactivation at a Post-Transcriptional Level
Rao et al. Retrovirology (2018) 15:42 https://doi.org/10.1186/s12977-018-0425-2 Retrovirology RESEARCH Open Access The RNA surveillance proteins UPF1, UPF2 and SMG6 afect HIV‑1 reactivation at a post‑transcriptional level Shringar Rao1,2, Raquel Amorim1,3, Meijuan Niu1, Abdelkrim Temzi1 and Andrew J. Mouland1,2,3* Abstract Background: The ability of human immunodefciency virus type 1 (HIV-1) to form a stable viral reservoir is the major obstacle to an HIV-1 cure and post-transcriptional events contribute to the maintenance of viral latency. RNA surveil- lance proteins such as UPF1, UPF2 and SMG6 afect RNA stability and metabolism. In our previous work, we dem- onstrated that UPF1 stabilises HIV-1 genomic RNA (vRNA) and enhances its translatability in the cytoplasm. Thus, in this work we evaluated the infuence of RNA surveillance proteins on vRNA expression and, as a consequence, viral reactivation in cells of the lymphoid lineage. Methods: Quantitative fuorescence in situ hybridisation—fow cytometry (FISH-fow), si/shRNA-mediated deple- tions and Western blotting were used to characterise the roles of RNA surveillance proteins on HIV-1 reactivation in a latently infected model T cell line and primary CD4 T cells. + Results: UPF1 was found to be a positive regulator of viral reactivation, with a depletion of UPF1 resulting in impaired vRNA expression and viral reactivation. UPF1 overexpression also modestly enhanced vRNA expression and its ATPase activity and N-terminal domain were necessary for this efect. UPF2 and SMG6 were found to negatively infuence viral reactivation, both via an interaction with UPF1.