The Organometallic Active Site of [Fe]Hydrogenase: Models And
Total Page:16
File Type:pdf, Size:1020Kb
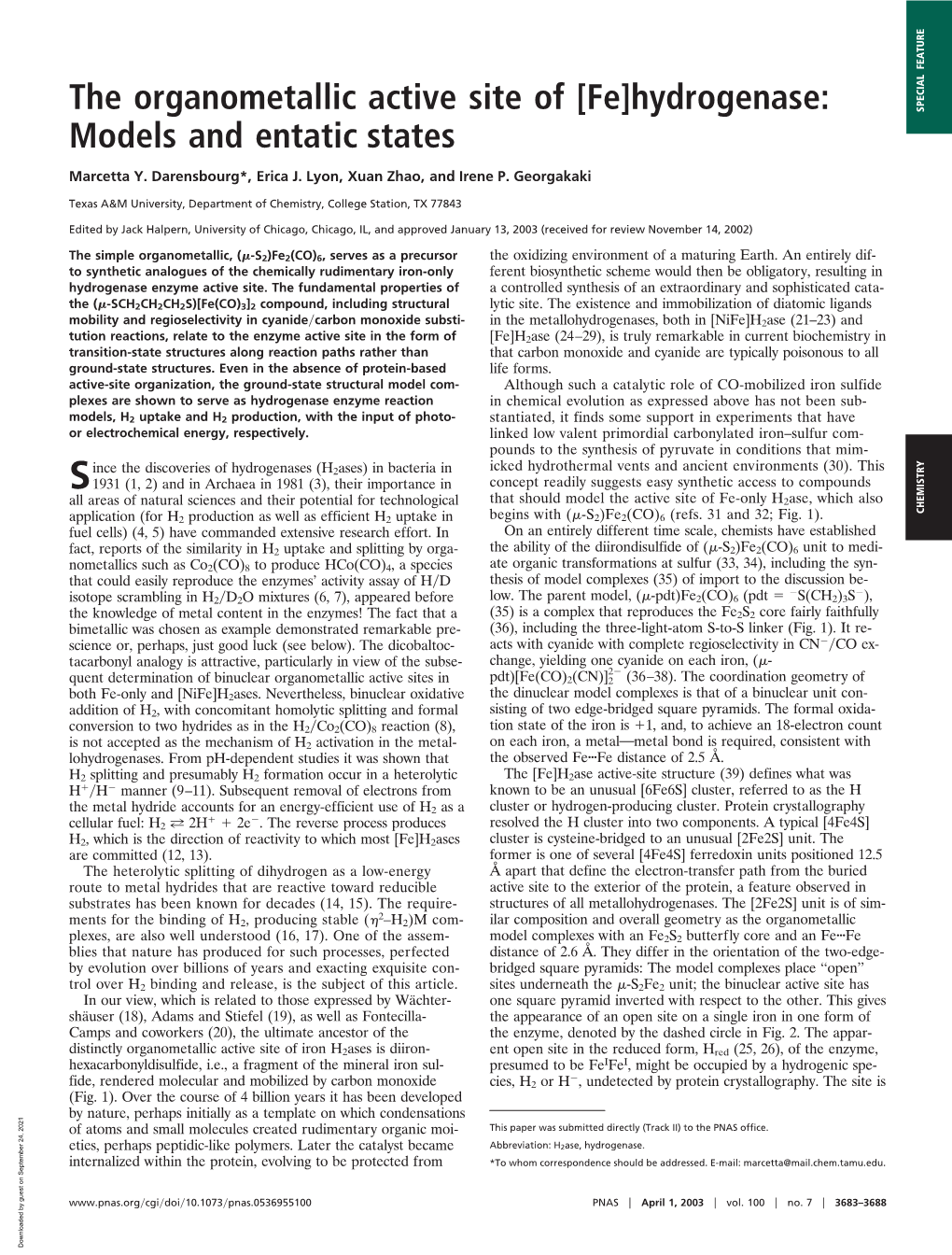
Load more
Recommended publications
-
A Web Tool for Hydrogenase Classification and Analysis Dan Søndergaard1, Christian N
www.nature.com/scientificreports OPEN HydDB: A web tool for hydrogenase classification and analysis Dan Søndergaard1, Christian N. S. Pedersen1 & Chris Greening2,3 H2 metabolism is proposed to be the most ancient and diverse mechanism of energy-conservation. The Received: 24 June 2016 metalloenzymes mediating this metabolism, hydrogenases, are encoded by over 60 microbial phyla Accepted: 09 September 2016 and are present in all major ecosystems. We developed a classification system and web tool, HydDB, Published: 27 September 2016 for the structural and functional analysis of these enzymes. We show that hydrogenase function can be predicted by primary sequence alone using an expanded classification scheme (comprising 29 [NiFe], 8 [FeFe], and 1 [Fe] hydrogenase classes) that defines 11 new classes with distinct biological functions. Using this scheme, we built a web tool that rapidly and reliably classifies hydrogenase primary sequences using a combination of k-nearest neighbors’ algorithms and CDD referencing. Demonstrating its capacity, the tool reliably predicted hydrogenase content and function in 12 newly-sequenced bacteria, archaea, and eukaryotes. HydDB provides the capacity to browse the amino acid sequences of 3248 annotated hydrogenase catalytic subunits and also contains a detailed repository of physiological, biochemical, and structural information about the 38 hydrogenase classes defined here. The database and classifier are freely and publicly available at http://services.birc.au.dk/hyddb/ Microorganisms conserve energy by metabolizing H2. Oxidation of this high-energy fuel yields electrons that can be used for respiration and carbon-fixation. This diffusible gas is also produced in diverse fermentation and 1 anaerobic respiratory processes . H2 metabolism contributes to the growth and survival of microorganisms across the three domains of life, including chemotrophs and phototrophs, lithotrophs and heterotrophs, aerobes and 1,2 anaerobes, mesophiles and extremophiles alike . -
Hydrogenases of Methanogens
ANRV413-BI79-18 ARI 27 April 2010 21:0 Hydrogenases from Methanogenic Archaea, Nickel, a Novel Cofactor, and H2 Storage Rudolf K. Thauer, Anne-Kristin Kaster, Meike Goenrich, Michael Schick, Takeshi Hiromoto, and Seigo Shima Max Planck Institute for Terrestrial Microbiology, D-35043 Marburg, Germany; email: [email protected] Annu. Rev. Biochem. 2010. 79:507–36 Key Words First published online as a Review in Advance on H2 activation, energy-converting hydrogenase, complex I of the March 17, 2010 respiratory chain, chemiosmotic coupling, electron bifurcation, The Annual Review of Biochemistry is online at reversed electron transfer biochem.annualreviews.org This article’s doi: Abstract 10.1146/annurev.biochem.030508.152103 Most methanogenic archaea reduce CO2 with H2 to CH4. For the Copyright c 2010 by Annual Reviews. activation of H2, they use different [NiFe]-hydrogenases, namely All rights reserved energy-converting [NiFe]-hydrogenases, heterodisulfide reductase- 0066-4154/10/0707-0507$20.00 associated [NiFe]-hydrogenase or methanophenazine-reducing by University of Texas - Austin on 06/10/13. For personal use only. [NiFe]-hydrogenase, and F420-reducing [NiFe]-hydrogenase. The energy-converting [NiFe]-hydrogenases are phylogenetically related Annu. Rev. Biochem. 2010.79:507-536. Downloaded from www.annualreviews.org to complex I of the respiratory chain. Under conditions of nickel limitation, some methanogens synthesize a nickel-independent [Fe]- hydrogenase (instead of F420-reducing [NiFe]-hydrogenase) and by that reduce their nickel requirement. The [Fe]-hydrogenase harbors a unique iron-guanylylpyridinol cofactor (FeGP cofactor), in which a low-spin iron is ligated by two CO, one C(O)CH2-, one S-CH2-, and a sp2-hybridized pyridinol nitrogen. -
A Web Tool for Hydrogenase Classification and Analysis
bioRxiv preprint doi: https://doi.org/10.1101/061994; this version posted September 16, 2016. The copyright holder for this preprint (which was not certified by peer review) is the author/funder, who has granted bioRxiv a license to display the preprint in perpetuity. It is made available under aCC-BY-NC-ND 4.0 International license. 1 HydDB: A web tool for hydrogenase 2 classification and analysis 3 Dan Søndergaarda, Christian N. S. Pedersena, Chris Greeningb, c* 4 5 a Aarhus University, Bioinformatics Research Centre, C.F. Møllers Allé 8, Aarhus 6 DK-8000, Denmark 7 b The Commonwealth Scientific and Industrial Research Organisation, Land and 8 Water Flagship, Clunies Ross Street, Acton, ACT 2060, Australia 9 c Monash University, School of Biological Sciences, Clayton, VIC 2800, Australia 10 11 Correspondence: 12 Dr Chris Greening ([email protected]), Monash University, School of 13 Biological Sciences, Clayton, VIC 2800, Australia 14 Dan Søndergaard ([email protected]), Aarhus University, Bioinformatics Research 15 Centre, C.F. Møllers Allé 8, Aarhus DK-8000, Denmark 1 bioRxiv preprint doi: https://doi.org/10.1101/061994; this version posted September 16, 2016. The copyright holder for this preprint (which was not certified by peer review) is the author/funder, who has granted bioRxiv a license to display the preprint in perpetuity. It is made available under aCC-BY-NC-ND 4.0 International license. 16 Abstract 17 H2 metabolism is proposed to be the most ancient and diverse mechanism of 18 energy-conservation. The metalloenzymes mediating this metabolism, 19 hydrogenases, are encoded by over 60 microbial phyla and are present in all major 20 ecosystems. -
Reconstitution of [Fe]-Hydrogenase Using Model Complexes
View metadata, citation and similar papers at core.ac.uk brought to you by CORE provided by Infoscience - École polytechnique fédérale de Lausanne Reconstitution of [Fe]-hydrogenase using model complexes Seigo Shima1,2,*, Dafa Chen3, Tao Xu4, Matthew D. Wodrich4,5, Takashi Fujishiro1, Katherine M. Schultz4, Jörg Kahnt1, Kenichi Ataka6 & Xile Hu4,* 1Max Planck Institute for Terrestrial Microbiology, 35043 Marburg, Germany. 2PRESTO, Japan Science and Technology Agency (JST), 332-0012 Saitama, Japan. 3School of Chemical Engineering and Technology, Harbin Institute of Technology, 150001 Harbin, China. 4Laboratory of Inorganic Synthesis and Catalysis, Institute of Chemical Science and Engineering, Ecole Polytechnique Fédérale de Lausanne (EPFL), 1015 Lausanne, Switzerland. 5Laboratory for Computational Molecular Design, Institute of Chemical Science and Engineering, Ecole Polytechnique Fédérale de Lausanne (EPFL), 1015 Lausanne, Switzerland. 6Department of Physics, Freie Universität Berlin, 14195 Berlin, Germany. 1 Abstract. [Fe]-Hydrogenase catalyzes the reversible hydrogenation of a methenyl- tetrahydromethanopterin substrate, which is an intermediate step during methanogenesis from CO2 and H2. The active site contains an iron-guanylylpyridinol (FeGP) cofactor, in which Fe2+ is coordinated by two CO ligands, as well as an acyl carbon atom and a pyridinyl nitrogen atom from a 3,4,5,6-substituted 2-pyridinol ligand. However, the mechanism of H2 activation by [Fe]-hydrogenase is unclear. Here, we report reconstitution of [Fe]-hydrogenase from an apoenzyme using two FeGP cofactor mimics to create semi-synthetic enzymes. The small molecule mimics reproduce the ligand environment of the active site, but are inactive towards H2 binding and activation on their own. We show that reconstituting the enzyme using a mimic containing a 2-hydroxy pyridine group restores activity, whilst an analogous experiment with a 2-methoxy-pyridine complex was essentially inactive. -
Perspectives
PERSPECTIVES in a number of enzymes such as [FeFe]- hydrogenase, [FeNi]-hydrogenase, Natural inspirations for metal–ligand [Fe]-hydrogenase, lactate racemase and alcohol dehydrogenase. In this Perspective, cooperative catalysis we discuss these examples of biological cooperative catalysis and how, inspired by these naturally occurring systems, chemists Matthew D. Wodrich and Xile Hu have sought to create functional analogues. Abstract | In conventional homogeneous catalysis, supporting ligands act as We also speculate that cooperative catalysis spectators that do not interact directly with substrates. However, in metal–ligand may be at play in [NiFe]-carbon monoxide cooperative catalysis, ligands are involved in facilitating reaction pathways that dehydrogenase ([NiFe]-CODHase), which may inspire the design of synthetic CO2 would be less favourable were they to occur solely at the metal centre. This reduction catalysts making use of these catalysis paradigm has been known for some time, in part because it is at play motifs. Each section in the following in enzyme catalysis. For example, studies of hydrogenative and dehydrogenative discussion is devoted to an enzyme and its enzymes have revealed striking details of metal–ligand cooperative catalysis synthetic models. that involve functional groups proximal to metal active sites. In addition to [FeFe]- and [NiFe]-hydrogenase the more well-known [FeFe]-hydrogenase and [NiFe]-hydrogenase enzymes, Hydrogenases are enzymes that catalyse [Fe]-hydrogenase, lactate racemase and alcohol dehydrogenase each makes use (REF. 5) the production and utilization of H2 . of cooperative catalysis. This Perspective highlights these enzymatic examples of Three variants of hydrogenase enzymes exist metal–ligand cooperative catalysis and describes functional bioinspired which are named [FeFe]-, [NiFe]- and [Fe]-hydrogenase because their active molecular catalysts that also make use of these motifs. -
Hydrogenase and Ferredoxin:NADP -Oxidoreductase (FNR)
Photosynthetic electron partitioning between [FeFe]- hydrogenase and ferredoxin:NADPþ-oxidoreductase (FNR) enzymes in vitro Iftach Yacobya,1, Sergii Pochekailova, Hila Toporikb, Maria L. Ghirardic, Paul W. Kingc,1, and Shuguang Zhanga,1 aCenter for Biomedical Engineering NE47-379, Massachusetts Institute of Technology, 77 Massachusetts Avenue, Cambridge, MA 02139-4307; cBiosciences Center, National Renewable Energy Laboratory, 1617 Cole Boulevard, Golden, CO 80401-3305; and bDepartment of Biochemistry and Molecular Biology, The George S. Wise Faculty of Life Sciences, Tel Aviv University, Tel Aviv, 69978, Israel Edited by Alan R. Fersht, Medical Research Council Laboratory of Molecular Biology, Cambridge, United Kingdom, and approved April 28, 2011 (receivedfor review March 5, 2011) Photosynthetic water splitting, coupled to hydrogenase-catalyzed hydrogen production, is considered a promising clean, renewable source of energy. It is widely accepted that the oxygen sensitivity of hydrogen production, combined with competition between hydrogenases and NADPH-dependent carbon dioxide fixation are the main limitations for its commercialization. Here we provide evi- dence that, under the anaerobic conditions that support hydrogen production, there is a significant loss of photosynthetic electrons toward NADPH production in vitro. To elucidate the basis for com- petition, we bioengineered a ferredoxin-hydrogenase fusion and characterized hydrogen production kinetics in the presence of Fd, ferredoxin:NADPþ-oxidoreductase (FNR), and NADPþ. Replacing the hydrogenase with a ferredoxin-hydrogenase fusion switched the bias of electron transfer from FNR to hydrogenase and resulted in an increased rate of hydrogen photoproduction. These results suggest a new direction for improvement of biohydrogen produc- tion and a means to further resolve the mechanisms that control partitioning of photosynthetic electron transport. -
Heterologous Hydrogenase Overproduction Systems for Biotechnology—An Overview
International Journal of Molecular Sciences Review Heterologous Hydrogenase Overproduction Systems for Biotechnology—An Overview Qin Fan 1 , Peter Neubauer 1 , Oliver Lenz 2 and Matthias Gimpel 1,* 1 Institute of Biotechnology, Technical University of Berlin, Ackerstraße 76, 13355 Berlin, Germany; [email protected] (Q.F.); [email protected] (P.N.) 2 Department of Chemistry, Technical University of Berlin, Straße des 17. Juni 135, 10623 Berlin, Germany; [email protected] * Correspondence: [email protected] Received: 14 July 2020; Accepted: 14 August 2020; Published: 16 August 2020 Abstract: Hydrogenases are complex metalloenzymes, showing tremendous potential as H2-converting redox catalysts for application in light-driven H2 production, enzymatic fuel cells and H2-driven cofactor regeneration. They catalyze the reversible oxidation of hydrogen into protons and electrons. The apo-enzymes are not active unless they are modified by a complicated post-translational maturation process that is responsible for the assembly and incorporation of the complex metal center. The catalytic center is usually easily inactivated by oxidation, and the separation and purification of the active protein is challenging. The understanding of the catalytic mechanisms progresses slowly, since the purification of the enzymes from their native hosts is often difficult, and in some case impossible. Over the past decades, only a limited number of studies report the homologous or heterologous production of high yields of hydrogenase. In this review, we emphasize recent discoveries that have greatly improved our understanding of microbial hydrogenases. We compare various heterologous hydrogenase production systems as well as in vitro hydrogenase maturation systems and discuss their perspectives for enhanced biohydrogen production. -
Genomic and Metagenomic Surveys of Hydrogenase Distribution Indicate H2 Is a Widely Utilised Energy Source for Microbial Growth and Survival
The ISME Journal (2016) 10, 761–777 © 2016 International Society for Microbial Ecology All rights reserved 1751-7362/16 www.nature.com/ismej ORIGINAL ARTICLE Genomic and metagenomic surveys of hydrogenase distribution indicate H2 is a widely utilised energy source for microbial growth and survival Chris Greening1,2, Ambarish Biswas1, Carlo R Carere3,4, Colin J Jackson5, Matthew C Taylor2, Matthew B Stott3, Gregory M Cook1,6 and Sergio E Morales1 1Department of Microbiology and Immunology, University of Otago, Dunedin, New Zealand; 2The Commonwealth Scientific and Industrial Research Organisation, Land and Water Flagship, Acton, Australian Capital Territory, Australia; 3GNS Science, Wairakei Research Centre, Taupō, New Zealand; 4Scion, Te Papa Tipu Innovation Park, Rotorua, New Zealand; 5Australian National University, Research School of Chemistry, Acton, Australian Capital Territory, Australia and 6University of Auckland, Maurice Wilkins Centre for Molecular Biodiscovery, Auckland, New Zealand Recent physiological and ecological studies have challenged the long-held belief that microbial metabolism of molecular hydrogen (H2) is a niche process. To gain a broader insight into the importance of microbial H2 metabolism, we comprehensively surveyed the genomic and metage- nomic distribution of hydrogenases, the reversible enzymes that catalyse the oxidation and evolution of H2. The protein sequences of 3286 non-redundant putative hydrogenases were curated from publicly available databases. These metalloenzymes were classified into multiple groups based on (1) amino acid sequence phylogeny, (2) metal-binding motifs, (3) predicted genetic organisation and (4) reported biochemical characteristics. Four groups (22 subgroups) of [NiFe]-hydrogenase, three groups (6 subtypes) of [FeFe]-hydrogenases and a small group of [Fe]-hydrogenases were identified. -
Hydrogenases of Methanococcus Maripaludis
Hydrogenases of Methanococcus maripaludis John A. Leigh, William B. Whitman, and Murray Hackett University of Washington Box 357242 Seattle, WA 98195-7242 206-685-1390, FAX 206-543-8297 [email protected] DOE Program Officer: Richard Greene [email protected]; 301 903 6190 Subcontractors: William B. Whitman, University of Georgia Objectives • The research examines the enzymes and pathways in H2 metabolism in methanogens, especially as they relate to energy conservation. In addition, the metabolism of formate, from which H2 is produced, is investigated. Technical Barriers • The research should lead to an enhanced understanding of how two universal forms of biological energy, the chemical energy contained in the bonds of molecular hydrogen and the energy stored in ion gradients that form across cell membranes, can be interconverted. Abstract The methanogens catalyze a major component of the Earth’s H2 cycle. They are especially active in anaerobic environments where they are the primary consumers of fermentatively produced H2. These strictly anaerobic Archaea have evolved unique adaptations to H2 metabolism, many of which are poorly understood. Our CO2 research examines the enzymes and F420H2 + + pathways in H2 metabolism in H or Na MFR Fdh methanogens, especially as they Fd (red) Fmd/ F420 (ox) Fwd Formate relate to energy conservation (Fig. Eha Fd (ox) 1). In addition, the metabolism of H2 Formyl-MFR H+ or Na+ formate, from which H2 is produced, H4MPT is being investigated. The model MFR Formyl-H4MPT species of choice is the H+ hydrogenotroph Methanococccus H2O Methenyl-H4MPT H2 maripaludis. Transcriptome and F420H2 Hmd + Mtd H + proteome studies have determined F420 (ox) + H F420H2 Methylene-H4MPT the regulatory effects of H2 F420H2 Fru/c H2 limitation. -
XXVII. HYDROGENASE: a BACTERIAL ENZYME ACTIVATING MOLECULAR HYDROGEN. Experimental
XXVII. HYDROGENASE: A BACTERIAL ENZYME ACTIVATING MOLECULAR HYDROGEN. I. THE PROPERTIES OF THE ENZYME. BY MARJORY STEPHENSON AND LEONARD HUBERT STICKLAND1. From the Biochemical Laboratory, Cambridge. (Received December 13th, 1930.) BIoCHEMISTs are now accustomed to regard the transfer of hydrogen as an essential step in biological oxidations; such a view involves the conception of some enzymic mechanism for rendering active or unstable the molecule from which the hydrogen is transferred. No such enzyme acting on molecular hydrogen has so far been described, though several inorganic catalysts are known which function in this way. It is nevertheless almost certain that such an enzyme exists, as organisms producing molecular hydrogen presumably have such a catalyst; moreover bacteria have long been known which are capable of oxidising hydrogen gas by molecular oxygen and living autotrophic- ally on the energy thus liberated. The earliest example of such an organism was B. pantotrophus (Hydrogenomonas pantotropha) isolated by Kaserer [1906]. Later other workers isolated a variety of species having the same characteristic [Niklewski, 1914; Grohmann, 1924]. It was subsequently discovered that in certain of these species nitrate can replace oxygen, so that an anaerobic auto- trophic development occurs [Niklewski, 1914]. In all these cases the organisms are facultatively autotrophic, the oxidation of hydrogen playing an essential part in their metabolism. A suggestion that these early observations come into line with recent work on the dehydrogenases of bacteria is contained in the recent work of Tausz and Donath [1930], who showed that a culture of Bact. aliphaticum lique- faciens grown autotrophically on hydrogen, oxygen and carbon dioxide is capable of reducing methylene blue when a stream of hydrogen is led through the apparatus, but not when nitrogen replaces the hydrogen. -
Genomic Characterization and Environmental Distribution of A
Supplementary material Genomic characterization and environmental distribution of a thermophilic anaerobe Dissulfurirhabdus thermomarina SH388T involved in disproportionation of sulfur compounds in shallow- sea hydrothermal vents Maxime Allioux 1, Stéven Yvenou 1, Galina Slobodkina 2, Alexander Slobodkin 2, Zongze Shao 3, Mohamed Jebbar 1 and Karine Alain 1,* 1 Univ Brest, CNRS, IFREMER, LIA1211, Laboratoire de Microbiologie des Environnements Extrêmes LM2E, IUEM, Rue Dumont d’Urville, F-29280 Plouzané, France; [email protected] (M.A.); [email protected] (S.Y.); [email protected] (M.J.); [email protected] (K.A.) 2 Winogradsky Institute of Microbiology, Research Center of Biotechnology of the Russian Academy of Sciences, 117312 Moscow, Russia; [email protected] (G.S.); [email protected] (A.S.) 3 Key Laboratory of Marine Genetic Resources, Third Institute of Oceanography, Ministry of Natural Resources, Xiamen 361005, China; [email protected] (Z.S.) * Correspondence: [email protected] Supplementary Material S1: Dissulfurirhabdus thermomarina SH388T genome locus tags, genomic islands composition, and results of comparative genomics. S1.1. Synthesis of the gene loci Table S1.1. Correspondences between the loci of the annotations by Prokka, Dfast, RAST, PGAP (2020-03- 30.build4489) and UniProtKB with the CDSs of the NCBI's online automated prokaryotic genome annotation pipeline. CDSs found with their associated loci, based on the assembly repository ASM1297923v1 (Abbreviation: NR, not -
[Nife]-Hydrogenase Maturation
TSpace Research Repository tspace.library.utoronto.ca [NiFe]-Hydrogenase Maturation Michael J. Lacasse and Deborah B. Zamble Version Post-print/accepted manuscript Citation Lacasse, M. J., & Zamble, D. B. (2016). [NiFe]-hydrogenase maturation. (published version) Biochemistry, 55(12), 1689-1701. Publisher’s Statement This document is the Accepted Manuscript version of a Published Work that appeared in final form in Biochemistry, copyright © American Chemical Society after peer review and technical editing by the publisher. To access the final edited and published work see 10.1021/acs.biochem.5b01328. How to cite TSpace items Always cite the published version, so the author(s) will receive recognition through services that track citation counts, e.g. Scopus. If you need to cite the page number of the author manuscript from TSpace because you cannot access the published version, then cite the TSpace version in addition to the published version using the permanent URI (handle) found on the record page. This article was made openly accessible by U of T Faculty. Please tell us how this access benefits you. Your story matters. Biochemistry This document is confidential and is proprietary to the American Chemical Society and its authors. Do not copy or disclose without written permission. If you have received this item in error, notify the sender and delete all copies. [NiFe]-Hydrogenase Maturation Journal: Biochemistry Manuscript ID bi-2015-01328c.R1 Manuscript Type: Current Topic/Perspective Date Submitted by the Author: n/a Complete List of Authors: Lacasse, Michael; University of Toronto, Chemistry Zamble, Deborah; University of Toronto, Chemistry; University of Toronto, Biochemistry ACS Paragon Plus Environment Page 1 of 41 Biochemistry 1 2 3 4 [NiFe]-Hydrogenase Maturation 5 6 7 8 9 10 1 1, 2 11 Michael J.