Observation of a Charge-Neutral Muon-Polaron Complex in Antiferromagnetic Cr2o3
Total Page:16
File Type:pdf, Size:1020Kb
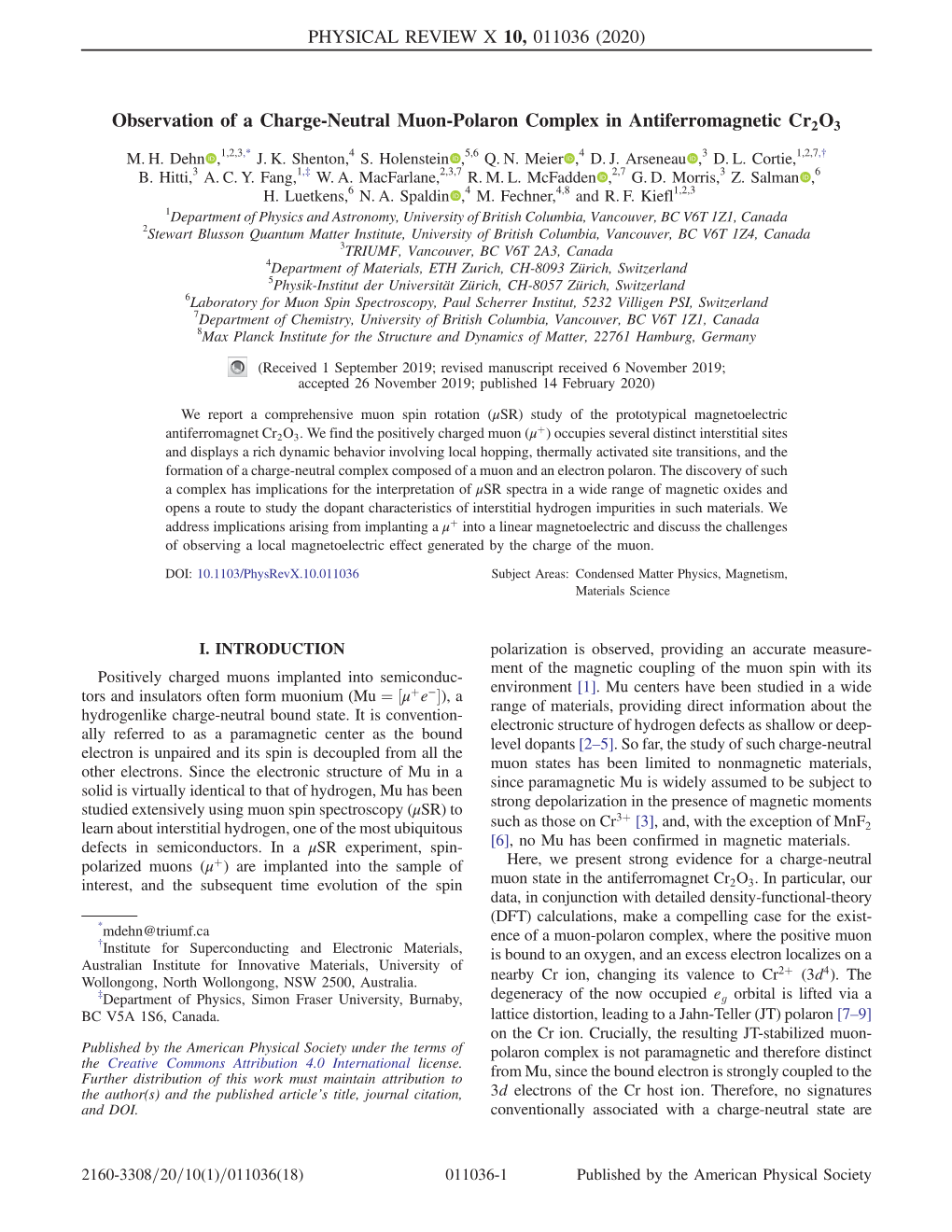
Load more
Recommended publications
-
Muon Spin Rotation/Relaxation/Resonance
Muon Spin µSR Rotation/Relaxation/Resonance (µµµSR) Unlike the electron, the muon is an magnetic moment is 3.18 times larger Understanding the fundamental unstable particle, living for only about than a proton. Thus when implanted in physical properties of matter at a two millionths of a second. Muons matter this feature makes them an microscopic level enables the extension carry a positive (µ+) or negative (µ-) extremely sensitive microscopic probe of existing technologies and leads to the charge, and spontaneously decay into a of magnetism. development of reliable new materials positron (or an electron) and a neutrino- for tomorrow’s applications. For these anti-neutrino pair as follows: reasons, scientists are interested in The Birth of µµµSR studying microscopic structures and µ e ++ ++→ νν processes in a wide range of materials. e µ he acronym µSR was coined in Only through experimental verification e −− ++→ ννµ T can one establish physical laws e µ 1974 to grace the cover of the first issue describing their inner workings. Since of the µSR Newsletter, in which the we cannot see such small-scale Muon following definition and explanation phenomena directly with our eyes, we were given: must rely on experimental methods that can probe deep inside materials for us. µµµSR stands for Muon Spin Relaxation, Rotation, Resonance, Research or The µSR technique is one such method. what have you. The intention of the It makes use of a short-lived subatomic mnemonic acronym is to draw particle called a muon, whose spin and attention to the analogy with NMR and charge are exquisitely sensitive local ESR, the range of whose applications magnetic and electronic probes of is well known. -
Future Muon Source Possibilities at the SNS
ORNL/TM-2017/165 Future Muon Source Possibilities at the SNS Gregory J. MacDougall Travis J. Williams Date: March 28, 2017 DOCUMENT AVAILABILITY Reports produced after January 1, 1996, are generally available free via US Department of Energy (DOE) SciTech Connect. Website http://www.osti.gov/scitech/ Reports produced before January 1, 1996, may be purchased by members of the public from the following source: National Technical Information Service 5285 Port Royal Road Springfield, VA 22161 Telephone 703-605-6000 (1-800-553-6847) TDD 703-487-4639 Fax 703-605-6900 E-mail [email protected] Website http://www.ntis.gov/help/ordermethods.aspx Reports are available to DOE employees, DOE contractors, Energy Technology Data Exchange representatives, and International Nuclear Information System representatives from the following source: Office of Scientific and Technical Information PO Box 62 Oak Ridge, TN 37831 Telephone 865-576-8401 Fax 865-576-5728 E-mail [email protected] Website http://www.osti.gov/contact.html This report was prepared as an account of work sponsored by an agency of the United States Government. Neither the United States Government nor any agency thereof, nor any of their employees, makes any warranty, express or implied, or assumes any legal liability or responsibility for the accuracy, completeness, or usefulness of any information, apparatus, product, or process disclosed, or represents that its use would not infringe privately owned rights. Reference herein to any specific commercial product, process, or service by trade name, trademark, manufacturer, or otherwise, does not necessarily constitute or imply its endorsement, recommendation, or favoring by the United States Government or any agency thereof. -
Hydrogen Impurity in Paratellurite Α-Teo2 Using Muon-Spin Rotation
Hydrogen impurity in paratellurite α-TeO2 using Muon-spin rotation Disserta¸c˜aosubmetida para a obten¸c˜aodo Grau de Mestre em F´ısica com especializa¸c˜aoem F´ısica da Mat´eria Condensada Ricardo Borda Lopes Vieira Orientador: Professor Doutor Rui C´esar do Esp´ırito Santo Vil˜ao Coimbra, 2011 Para a Tˆania OGabriel EosmeusPais Abstract We have investigated the behavior of isolated hydrogen in paratellurite (α-TeO2)bymeansofmuon-spinrotationspectroscopy(µSR) measurements. The observable metastable states accessible by means of the muon implan- tation allowed us to probe both the donor and the acceptor configurations of hydrogen, as well as to follow their dynamics. A shallow donor state with an ionization energy of 6 meV, as well as a deep acceptor state are proposed. From the experimental µSR results, the donor (+/0) conversion level was located near the conduction band edge, consistent with DFT results. Atom- like interstitial muonium was also observed; it has a hyperfine interaction of about 3.5 GHz, possibly slightly anisotropic. Possible site exchange (deep to shallow conversion) and charge exchange high temperature dynamics are discussed, and a dynamical model for the formation of Mu− is discussed and simulated. Preliminary work on yttrium oxide (Y2O3)isalsopresented,aswellas, possible hydrogen configurations. Resumo Investig´amos o comportamento de hidrog´enio isolados em paratellurite (α-TeO2), por meio de medi¸c˜oes espectroscopia de mu˜aopositivo (µSR). Os estados metaest´aveis observ´aveis acess´ıveis por meio da implanta¸c˜ao do mu˜ao permitiram-nos sondar as configura¸c˜oes dadora e aceitadora do hidrog´enio, bem como acompanhar a sua dinˆamica. -
Muon Spin Spectroscopy Study of the Noncentrosymmetric
Superconducting and normal state properties of noncentrosymmetric superconductor NbOs2 investigated by muon spin relaxation and rotation D. Singh,1 Sajilesh K.P,1 Sourav Marik,1 A. D. Hillier,2 and R. P. Singh1, ∗ 1Indian Institute of Science Education and Research Bhopal, Bhopal, 462066, India 2ISIS facility, STFC Rutherford Appleton Laboratory, Harwell Science and Innovation Campus, Oxfordshire, OX11 0QX, UK (Dated: January 18, 2019) Noncentrosymmetric superconductors with α-manganese structure has attracted much attention re- cently, after the discovery of time-reversal symmetry breaking in all the members of Re6X (X = Ti, Hf, Zr) family. Similar to Re6X, NbOs2 also adopts α-Mn structure and found to be supercon- ducting with critical temperature Tc ' 2.7 K. The results of the resistivity, magnetization, specific heat and muon-spin relaxation/rotation measurements show that NbOs2 is a weakly coupled type- II superconductor. Interestingly, the zero-field muon experiments indicate that the time-reversal symmetry is preserved in the superconducting state. The low-temperature transverse-field muon measurements and the specific heat data evidence an conventional isotropic fully gapped super- conductivity. However, the calculated electronic properties in this material show that the NbOs2 is positioned close to the band of unconventionality of the Uemura plot, indicating that NbOs2 potentially borders an unconventional superconducting ground state. I. INTRODUCTION In these superconductors, additional complications come due to the presence of strong electronic correlation effects The discovery of exotic superconducting properties in and quantum criticality, which often hinders the research the heavy fermion noncentrosymmetric superconductor aimed to understand the interplay between crystal sym- (NCS) CePt3Si [1], sparked renewed research interest metry and superconductivity. -
A Low Energy Muon Spin Rotation and Point Contact Tunneling Study of Niobium films Prepared for Superconducting Cavities
A low energy muon spin rotation and point contact tunneling study of niobium films prepared for superconducting cavities Tobias Junginger∗ Helmholtz-Zentrum Berlin fuer Materialien und Energie (HZB), Germanyy S. Calatroni, A. Sublet, and G. Terenziani European Organisation for Nuclear Research (Cern),Geneva Switzerland T. Prokscha, Z. Salman, and A. Suter Paul Scherrer Institut (PSI), Villigen, Switzerland T. Proslier Argonne National Laboratory, USA and Commissariat de l'´energie atomique et aux ´energies renouvelables, France J. Zasadzinski Illinois Institute of Technology, Chicago, USA (Dated: April 11, 2018) Point contact tunneling (PCT) and low energy muon spin rotation (LE-µSR) are used to probe, on the same samples, the surface superconducting properties of micrometer thick niobium films deposited onto copper substrates using different sputtering techniques: diode, dc magnetron (dcMS) and HIPIMS. The combined results are compared to radio-frequency tests performances of RF cavities made with the same processes. Degraded surface superconducting properties are found to correlate to lower quality factors and stronger Q slope. In addition, both techniques find evidence for surface paramagnetism on all samples and particularly on Nb films prepared by HIPIMS. I. CURRENT LIMITATIONS OF NIOBIUM ON hypotheses need to be addressed individually to identify COPPER CAVITIES their origin and possibly mitigate their influence on the surface impedance and SRF cavity performances. Superconducting cavities prepared by coating a mi- crometer thick niobium film on a copper substrate enable a lower surface resistance compared to bulk niobium at 4.5 K the operation temperature of several accelerators A. Surface magnetism, a possible source of RF using this technology, like the LHC or the HIE-Isolde dissipation at CERN. -
Paul Scherrer Institut Thomas Prokscha, Laboratory for Muon Spin Spectroscopy
Wir schaffen Wissen – heute für morgen Paul Scherrer Institut Thomas Prokscha, Laboratory for Muon Spin Spectroscopy The PSI continuous muon beam and low-energy µSR facilities ISIS Muon Spectroscopy Training School, March 21, 2012 RAL/ISIS PSI EL issF Sw n SINQ µSµS γ SLS PSI proton accelerator complex SINQ, neutron spallation source Dolly, µSR, GPS clone Low-energy muon beam facility (1-30 keV), LEM GPD, high pressure µSR High-Field (9.5 T, 20 mK) µSR, 2013 GPS/LTF, general purpose and low T (20 mK) µSR facility Ultra-cold neutrons UCN proton therapy, irradiation facility 50 MHz proton cyclotron, 2.2 mA, 590 MeV, 1.3 MW beam power (2.4 mA, 1.4 MW test operation); Comet cyclotron (superconducting), most powerful cyclotron worldwide and most 250 MeV, 500 nA, 72.8 MHz intense muon beams (quasi-continuous); The PSI isochronous cyclotron 2.2 mA: ~1.4 x 1016 protons/sec @ 590 MeV (1.3 MW on 5x5 mm2 = 50 kW/mm2, stainless steel melts in ~0.1 ms; electric power demand of 1000 households) A MW proton beam allows to generate 100% polarized 4-MeV µ+ beams with rates >108/sec Larmor frequency of protons: q/(2πm) = 15.25 MHz/T ν = q/(2πγm)∙B, γ = E /mc2 0 tot ν = n∙ν , frequency of accelerating radio-frequency rf 0 Isochronous cyclotron: B (R) ~ γ(R), constant ν ! 0 rf PSI cyclotron: B = 0.554 T, ν = 8.45 MHz, n = 6, 0 0 → ν = 50.7 MHz rf “Decay” and “Surface” muon beams Jess Brewer, UBC & TRIUMF: PSI muon beam time structure Pion decay time of 26 ns is “smearing” the proton beam structure in the muon beam. -
Muon Spin Resonance Analysis of the Internal Magnetic Field of Uranium Beryllium 13 Jack Li
Muon Spin Resonance Analysis of the Internal Magnetic Field of Uranium Beryllium 13 Jack Li To cite this version: Jack Li. Muon Spin Resonance Analysis of the Internal Magnetic Field of Uranium Beryllium 13 . 2017. hal-01536617v1 HAL Id: hal-01536617 https://hal.archives-ouvertes.fr/hal-01536617v1 Preprint submitted on 12 Jun 2017 (v1), last revised 5 May 2018 (v5) HAL is a multi-disciplinary open access L’archive ouverte pluridisciplinaire HAL, est archive for the deposit and dissemination of sci- destinée au dépôt et à la diffusion de documents entific research documents, whether they are pub- scientifiques de niveau recherche, publiés ou non, lished or not. The documents may come from émanant des établissements d’enseignement et de teaching and research institutions in France or recherche français ou étrangers, des laboratoires abroad, or from public or private research centers. publics ou privés. µSR ANALYSIS OF THE INTERNAL MAGNETIC FIELD OF UBe13 JACK LI Uranium beryllium 13 (UBe13) is a heavy fermion system whose properties depend strongly on its internal magnetic structure. Different models have been proposed to explain its magnetic distri- bution, but additional experimental data is required. An experimental method that is particularly useful is muon spin spectroscopy (µSR). In this process, positive muons are embedded into a sample where they localize at magnetically unique sites. The net magnetic field causes precession of the muon spin at the Larmor frequency, generating signals that can provide measurements of the internal field. This experiment specifically determines the muon localization sites of UBe13. To do so, results from muon spin experiments at various temperatures and external magnetic field strengths are an- alyzed. -
Muon Spin Rotation (Μsr) Technique and Its Applications in Magnetism and Superconductivity
Wir schaffen Wissen – heute für morgen Muon Spin Rotation (µSR) technique and its applications in magnetism and superconductivity Zurab Guguchia Laboratory for Muon Spin Spectroscopy Paul Scherrer Institut Muon is a Local Magnetic Probe Muon probes the local magnetism Muon probes the local magnetic from within the unit cell response of a superconductor (Meissner screening or flux line lattice) + ~Å ~100nm Zurab Guguchia Outline 1. Muon Properties – Pion decay – Muon decay – Parity violation – Muon spin precession 2. Muon Spin Rotation / Relaxation (SR) – Facilities around the world – Muon production at PSI – SR instruments at PSI – SR principle – Muon thermalization / Muon stopping sites / Muon stopping ranges – Measurement geometries 3. Muon Spin Rotation / Relaxation on Magnetic Materials – Different static depolarization functions and examples – Magnetic phase separation / coexistence of different magnetic phases – Magnetic fluctuations 4. Muon Spin Rotation on Superconducting Materials – Using low energy µSR to study the Meissner state of superconductors – Using bulk µSR to study the Vortex state of superconductors – Superfluid density and the symmetry of the superconducting gap – Magnetic and superconducting phase diagrams of Fe-based materials 5. Summary ZurabZurab Guguchia Guguchia Muon Properties Zurab Guguchia What is a Muon? Cosmics Muon Flux at sea level: ~ 1 Muon/Minute/cm2 Mean Energy: ~ 2 GeV Zurab Guguchia Muon Properties Elementary particle/antiparticle: mass: 200 x electron mass (105.6MeV/c2) 1/9 x proton mass charge: + e, oder - e spin: 1/2 magnetic moment : 3.18 x µp (8.9 x µN ), g 2.00 gyromagnetic ratio: 85.145 kHz/G unstable particle: mean lifetime: 2.2 µs N(t) = N(0)exp(-t/) Zurab Guguchia Muon production and polarised beams Pions as intermediate particles Protons of 600 to 800 MeV kinetic energy interact with protons or neutrons of the nuclei of a light element target to produce pions. -
Design of a Surface Muon Beam Line for High Field Musr at the PSI
TUPPC035 Proceedings of IPAC2012, New Orleans, Louisiana, USA DESIGN OF A SURFACE MUON BEAM LINE FOR HIGH FIELD mu SR AT THE PSI PROTON ACCELERATOR FACILITY K. Deiters, P. Kaufmann, T. Prokscha, T. Rauber, D. Reggiani#, R. Scheuermann, K. Sedlak, V. Vranković, Paul Scherrer Institut, 5232 Villigen PSI, Switzerland Y. Lee, European Spallation Source ESS AB, 221 00 Lund, Sweden Abstract proton accelerator, which provides a high intensity Starting from 2012, a High Field μSR (muon spin longitudinally polarized surface muon beam, has been rotation/relaxation/resonance) facility will come into permanently devoted to this new installation. For this operation in the πE3 secondary beam line located at the purpose, the second half of the beam line was completely target station E of the PSI proton accelerator. For this redesigned with the insertion of two new so-called spin purpose, the last part of the beam line was redesigned in rotator devices providing a virtually fully transversely order to integrate two electrostatic spin rotator devices polarized beam at the location of the sample material. providing a 90° rotation of the muon spin. At the same Simulations were performed in order to optimize the time, requirements of small beam diameter (σx,y ≈ 15 mm) beam transmission through the spin rotator system as well as well as small momentum bite (Δp/p ≈ 2 %) in the as the beam spot size at the probe location and to study, at sample region have to be met. This work focuses on the the same time, the dependence of the latter on the simulation of the beam optics (27.4 MeV/c design magnitude of the large magnetic field provided by the momentum). -
Μsr: Muon Spin Resonance
Owned and operated as a joint venture by a consortium of Canadian universities via a contribution through the National Research Council Canada History of A science fiction adventure story by Jess H. Brewer 2019 June 3-7 at Simon Fraser U. Congress of the Canadian Association of Physicists - History of Physics (DHP) 1 Owned and operated as a joint venture by a consortium of Canadian universities via a contribution through the National Research Council Canada History of 2019 June 3-7 at Simon Fraser U. Congress of the Canadian Association of Physicists - History of Physics (DHP) 1 OUTLINE Early History of µSR (“science fiction”?) Research “Themes” in µSR Development of Advanced Muon Beams µSR techniques (green: not invented at TRIUMF) acronyms: wTF, ZF, LF, Strobo, FFT, SR, HTF, RRF, ALCR, RF, μSI, E-field (µSR applications interleaved among techniques...) 2019 June 3-7 at Simon Fraser U. Congress of the Canadian Association of Physicists - History of Physics (DHP) 1 Evolution of μSR : Fantasy ➜ Fiction ➜ Physics 2019 June 3-7 at Simon Fraser U. Congress of the Canadian Association of Physicists - History of Physics (DHP) !3 Evolution of μSR : Fantasy ➜ Fiction ➜ Physics Fantasy: violates the “known laws of physics” 2019 June 3-7 at Simon Fraser U. Congress of the Canadian Association of Physicists - History of Physics (DHP) !3 Evolution of μSR : Fantasy ➜ Fiction ➜ Physics Fantasy: violates the “known laws of physics” Science Fiction: possible in principle, but impractical with existing technology. (Clarke’s Law: “Any sufficiently advanced technology is indistinguishable from magic.” ) 2019 June 3-7 at Simon Fraser U. -
Muon Spin Spectroscopy: Magnetism, Soft Matter and the Bridge Between the Two
TOPICAL REVIEW Muon spin spectroscopy: magnetism, soft matter and the bridge between the two L Nuccio1, L Schulz2;3, A J Drew3;4 1 Department of Physics and FriMAT, University of Fribourg, Chemin du Mus´ee 3, Fribourg CH-1700, Switzerland 2 Microelectronics Research Center, The University of Texas at Austin, 10100 Burnet Road, Blg. 160 Austin, Texas 78758, USA 3 Sichuan University, College of Physical Science & Technology, Chengdu 610064, Peoples Republic of China 4 School of Physics and Astronomy, Queen Mary University of London, Mile End Road, London E1 4NS, UK E-mail: [email protected] or [email protected] Abstract. The use of implanted muons to probe the spin dynamics and electronic excitations in a variety of magnetic and non-magnetic materials is reviewed and is split into three main sections, the first of which is an introduction to the historical context and background of the muon technique, which includes a basic introduction to the experimental method and underlying theoretical models. The second section is concerned with inorganic magnetic systems, starting with an overview of spin dynamics around critical points in ordered magnets. This is followed by an introduction to the early work on spin glasses, liquids and ices, which then continues onto the recent research in this area, including a discussion of some of the more controversial recent work on spin ices and magnetic monopoles. Information obtained by muons vital to two very important technological areas - magnetic semiconductors and next-generation energy materials - closes the discussion of inorganic magnetic materials. The final section is concerned with spin dynamics and magnetism in soft materials, and starts with discussing many of the key results in molecular magnets and organic spintronics. -
Quantum Spin-Liquid States in an Organic Magnetic Layer And
Quantum spin-liquid states in an organic magnetic layer and molecular rotor hybrid Peter´ Szirmaia ,Cecile´ Mezi´ ere` b, Guillaume Bastienc , Pawel Wzietekd , Patrick Batailb , Edoardo Martinoa, Konstantins Mantulnikovsa, Andrea Pisonia, Kira Riedle , Stephen Cottrellf, Christopher Bainesg ,Laszl´ o´ Forro´ a,1, and Balint´ Nafr´ adi´ a,1 aLaboratory of Physics of Complex Matter, Ecole Polytechnique Fed´ erale´ de Lausanne, CH-1015 Lausanne, Switzerland, 1015; bLaboratoire MOLTECH-Anjou, CNRS and Universite´ d’Angers, 49045 Angers, France, 49045 Angers; cInstitute of Organic Chemistry and Biochemistry, Academy of Science of the Czech Republic, 166 10 Prague 6, Czech Republic; dLaboratoire de Physique des Solides, CNRS and Universite´ de Paris-Sud, 91405 Orsay, France, 91405; eInstitut fur Theoretische Physik, Goethe-Universitat Frankfurt, 60438 Frankfurt am Main, Germany; fISIS Muon Group, Science and Technology Facilities Council (STFC), Didcot OX11 0QX, United Kingdom; and gLaboratory for Muon Spin Spectroscopy, Paul Scherrer Institute, 5232 Villigen, Switzerland Edited by Stuart Brown, University of California, Los Angeles, CA, and accepted by Editorial Board Member Zachary Fisk September 30, 2020 (received for review January 6, 2020) The exotic properties of quantum spin liquids (QSLs) have continu- tion and quenched bond randomness to induce or support a QSL ally been of interest since Anderson’s 1973 ground-breaking idea. state (23–25). It came as a surprise that the X-ray irradiation Geometrical frustration, quantum fluctuations, and low dimen- of the weakly frustrated, long-range ordered antiferromagnet, sionality are the most often evoked material’s characteristics κ-(ET)2Cu[N(CN)2]Cl was reported to lead to the stabilization that favor the long-range fluctuating spin state without freez- of a disorder-driven QSL state (26).