Common Signalling Pathways in Macrophage and Osteoclast Multinucleation
Total Page:16
File Type:pdf, Size:1020Kb
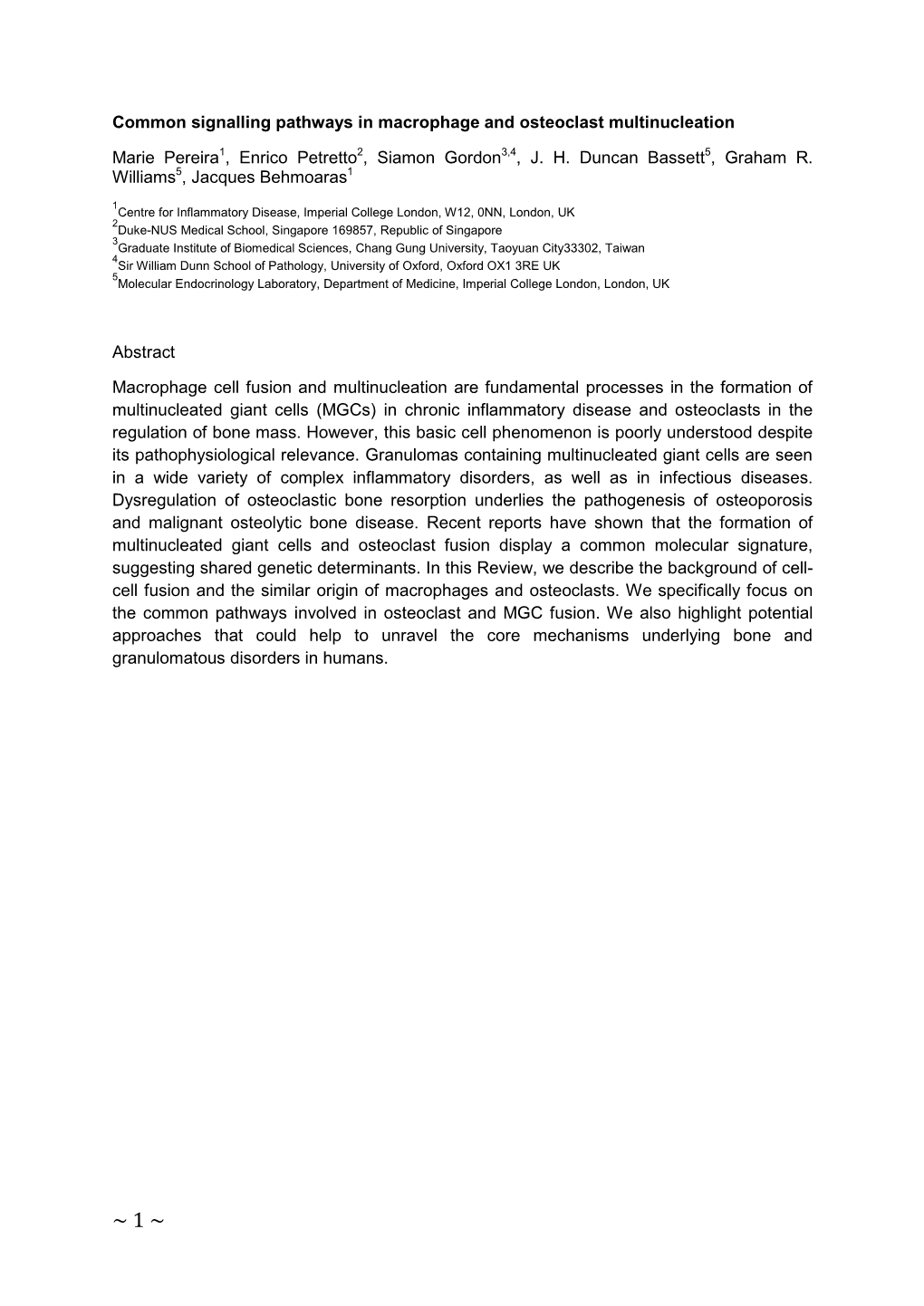
Load more
Recommended publications
-
ENSG Gene Encodes Effector TCR Pathway Costimulation Inhibitory/Exhaustion Synapse/Adhesion Chemokines/Receptors
ENSG Gene Encodes Effector TCR pathway Costimulation Inhibitory/exhaustion Synapse/adhesion Chemokines/receptors ENSG00000111537 IFNG IFNg x ENSG00000109471 IL2 IL-2 x ENSG00000232810 TNF TNFa x ENSG00000271503 CCL5 CCL5 x x ENSG00000139187 KLRG1 Klrg1 x ENSG00000117560 FASLG Fas ligand x ENSG00000121858 TNFSF10 TRAIL x ENSG00000134545 KLRC1 Klrc1 / NKG2A x ENSG00000213809 KLRK1 Klrk1 / NKG2D x ENSG00000188389 PDCD1 PD-1 x x ENSG00000117281 CD160 CD160 x x ENSG00000134460 IL2RA IL-2 receptor x subunit alpha ENSG00000110324 IL10RA IL-10 receptor x subunit alpha ENSG00000115604 IL18R1 IL-18 receptor 1 x ENSG00000115607 IL18RAP IL-18 receptor x accessory protein ENSG00000081985 IL12RB2 IL-12 receptor x beta 2 ENSG00000186810 CXCR3 CXCR3 x x ENSG00000005844 ITGAL CD11a x ENSG00000160255 ITGB2 CD18; Integrin x x beta-2 ENSG00000156886 ITGAD CD11d x ENSG00000140678 ITGAX; CD11c x x Integrin alpha-X ENSG00000115232 ITGA4 CD49d; Integrin x x alpha-4 ENSG00000169896 ITGAM CD11b; Integrin x x alpha-M ENSG00000138378 STAT4 Stat4 x ENSG00000115415 STAT1 Stat1 x ENSG00000170581 STAT2 Stat2 x ENSG00000126561 STAT5a Stat5a x ENSG00000162434 JAK1 Jak1 x ENSG00000100453 GZMB Granzyme B x ENSG00000145649 GZMA Granzyme A x ENSG00000180644 PRF1 Perforin 1 x ENSG00000115523 GNLY Granulysin x ENSG00000100450 GZMH Granzyme H x ENSG00000113088 GZMK Granzyme K x ENSG00000057657 PRDM1 Blimp-1 x ENSG00000073861 TBX21 T-bet x ENSG00000115738 ID2 ID2 x ENSG00000176083 ZNF683 Hobit x ENSG00000137265 IRF4 Interferon x regulatory factor 4 ENSG00000140968 IRF8 Interferon -
Human and Mouse CD Marker Handbook Human and Mouse CD Marker Key Markers - Human Key Markers - Mouse
Welcome to More Choice CD Marker Handbook For more information, please visit: Human bdbiosciences.com/eu/go/humancdmarkers Mouse bdbiosciences.com/eu/go/mousecdmarkers Human and Mouse CD Marker Handbook Human and Mouse CD Marker Key Markers - Human Key Markers - Mouse CD3 CD3 CD (cluster of differentiation) molecules are cell surface markers T Cell CD4 CD4 useful for the identification and characterization of leukocytes. The CD CD8 CD8 nomenclature was developed and is maintained through the HLDA (Human Leukocyte Differentiation Antigens) workshop started in 1982. CD45R/B220 CD19 CD19 The goal is to provide standardization of monoclonal antibodies to B Cell CD20 CD22 (B cell activation marker) human antigens across laboratories. To characterize or “workshop” the antibodies, multiple laboratories carry out blind analyses of antibodies. These results independently validate antibody specificity. CD11c CD11c Dendritic Cell CD123 CD123 While the CD nomenclature has been developed for use with human antigens, it is applied to corresponding mouse antigens as well as antigens from other species. However, the mouse and other species NK Cell CD56 CD335 (NKp46) antibodies are not tested by HLDA. Human CD markers were reviewed by the HLDA. New CD markers Stem Cell/ CD34 CD34 were established at the HLDA9 meeting held in Barcelona in 2010. For Precursor hematopoetic stem cell only hematopoetic stem cell only additional information and CD markers please visit www.hcdm.org. Macrophage/ CD14 CD11b/ Mac-1 Monocyte CD33 Ly-71 (F4/80) CD66b Granulocyte CD66b Gr-1/Ly6G Ly6C CD41 CD41 CD61 (Integrin b3) CD61 Platelet CD9 CD62 CD62P (activated platelets) CD235a CD235a Erythrocyte Ter-119 CD146 MECA-32 CD106 CD146 Endothelial Cell CD31 CD62E (activated endothelial cells) Epithelial Cell CD236 CD326 (EPCAM1) For Research Use Only. -
3034.Full.Pdf
Characterization of the CD200 Receptor Family in Mice and Humans and Their Interactions with CD200 This information is current as Gavin J. Wright, Holly Cherwinski, Mildred Foster-Cuevas, of September 28, 2021. Gary Brooke, Michael J. Puklavec, Mike Bigler, Yaoli Song, Maria Jenmalm, Dan Gorman, Terri McClanahan, Man-Ru Liu, Marion H. Brown, Jonathon D. Sedgwick, Joseph H. Phillips and A. Neil Barclay J Immunol 2003; 171:3034-3046; ; Downloaded from doi: 10.4049/jimmunol.171.6.3034 http://www.jimmunol.org/content/171/6/3034 References This article cites 39 articles, 20 of which you can access for free at: http://www.jimmunol.org/ http://www.jimmunol.org/content/171/6/3034.full#ref-list-1 Why The JI? Submit online. • Rapid Reviews! 30 days* from submission to initial decision • No Triage! Every submission reviewed by practicing scientists by guest on September 28, 2021 • Fast Publication! 4 weeks from acceptance to publication *average Subscription Information about subscribing to The Journal of Immunology is online at: http://jimmunol.org/subscription Permissions Submit copyright permission requests at: http://www.aai.org/About/Publications/JI/copyright.html Email Alerts Receive free email-alerts when new articles cite this article. Sign up at: http://jimmunol.org/alerts The Journal of Immunology is published twice each month by The American Association of Immunologists, Inc., 1451 Rockville Pike, Suite 650, Rockville, MD 20852 Copyright © 2003 by The American Association of Immunologists All rights reserved. Print ISSN: 0022-1767 Online ISSN: 1550-6606. The Journal of Immunology Characterization of the CD200 Receptor Family in Mice and Humans and Their Interactions with CD2001 Gavin J. -
CD200 and Its Receptor, CD200R, Modulate Bone Mass Via the Differentiation of Osteoclasts
CD200 and its receptor, CD200R, modulate bone mass via the differentiation of osteoclasts Weiguo Cui*†, Esteban Cuartas*, Juan Ke*‡, Qing Zhang*, Halldor B. Einarsson*, Jonathon D. Sedgwick§¶, Jun Liʈ, and Agne` s Vignery*,** *Department of Orthopedics and Rehabilitation, Yale School of Medicine, 310 Cedar Street, New Haven, CT 06510; §Schering–Plough Biopharma (DNAX), Palo Alto, CA 94304; and ʈDepartment of Immunology and Inflammation, Boehringer Ingelheim Pharmaceuticals, Inc., Ridgefield, CT 06877 Edited by Richard A. Flavell, Yale University School of Medicine, New Haven, CT, and approved July 25, 2007 (received for review April 11, 2007) Fusion of macrophages is an essential step in the differentiation of plays a role in the recognition of self and in the fusion of macro- osteoclasts, which play a central role in the development and remod- phages (10). To gain further insight into the mechanism of mac- eling of bone. Osteoclasts are important mediators of bone loss, rophage fusion, we subjected fusing alveolar macrophages from rats which leads, for example, to osteoporosis. Macrophage fusion recep- to genome-wide oligonucleotide microarray analysis, and we dis- tor/signal regulatory protein ␣ (MFR/SIRP␣) and its ligand CD47, covered the expression of CD200 de novo at the onset of fusion. which are members of the Ig superfamily (IgSF), have been implicated CD200 also belongs to the IgSF and has a short cytoplasmic tail. in the fusion of macrophages. We show that CD200, which is not It is expressed on various types of mouse and human cells (see ref. expressed in cells that belong to the myeloid lineage, is strongly 11 for a review) and on mouse osteoblasts (12), but not on expressed in macrophages at the onset of fusion. -
The Best Diagnosis Is: A
DErmatopathology Diagnosis The best diagnosis is: a. eruptive xanthomacopy H&E, original magnification ×200. b. juvenile xanthogranuloma c. Langerhans cell histiocytosis d. reticulohistiocytomanot e. Rosai-Dorfman disease Do CUTIS H&E, original magnification ×600. PLEASE TURN TO PAGE 39 FOR DERMATOPATHOLOGY DIAGNOSIS DISCUSSION Alyssa Miceli, DO; Nathan Cleaver, DO; Amy Spizuoco, DO Dr. Miceli is from the College of Osteopathic Medicine, New York Institute of Technology, Old Westbury. Drs. Cleaver and Spizuoco are from Ackerman Academy of Dermatopathology, New York, New York. The authors report no conflict of interest. Correspondence: Amy Spizuoco, DO, Ackerman Academy of Dermatopathology, 145 E 32nd Street, 10th Floor, New York, NY 10016 ([email protected]). 16 CUTIS® WWW.CUTIS.COM Copyright Cutis 2015. No part of this publication may be reproduced, stored, or transmitted without the prior written permission of the Publisher. Dermatopathology Diagnosis Discussion rosai-Dorfman Disease osai-Dorfman disease (RDD), also known as negative staining for CD1a on immunohistochemis- sinus histiocytosis with massive lymphade- try. Lymphocytes and plasma cells often are admixed nopathy, is a rare benign histioproliferative with the Rosai-Dorfman cells, and neutrophils and R 1 4 disorder of unknown etiology. Clinically, it is most eosinophils also may be present in the infiltrate. frequently characterized by massive painless cervical The histologic hallmark of RDD is emperipolesis, lymphadenopathy with other systemic manifesta- a phenomenon whereby inflammatory cells such as tions, including fever, night sweats, and weight loss. lymphocytes and plasma cells reside intact within Accompanying laboratory findings include leukocyto- the cytoplasm of histiocytes (Figure 2).5 sis with neutrophilia, elevated erythrocyte sedimenta- The histologic differential diagnosis of cutaneous tion rate, and polyclonal hypergammaglobulinemia. -
Lesmono Bayu, Dewi Yussy Afriani, Ratunanda Sinta Sari, Aroeman Nur Akbar
Necrobiotic Xanthogranuloma Sucessfully Treated with Cyclosphospamide-Methyl Prednisolon Lesmono Bayu, Dewi Yussy Afriani, Ratunanda Sinta Sari, Aroeman Nur Akbar Departement of Otorhinolaryngology Head and Neck Surgery Faculty of Medicine Padjadjaran University-Dr. Hasan Sadikin General Hospital Bandung ABSTRACT Objective: Necrobiotic Xanthogranuloma (NXG) is a rare, chronic, and progressive disease that provokes skin lesions, such as damage of the histiocytes of Non-Langerhans cell, skin lesions (yellowish or noduled ulcerative lesions) in the induration skin. The most common predilection areas are on the face, orbital, and extremities. The etiology is still unknown, but sometimes is often associated with monoclonal gammopathy. The granulomatous infiltrate was composed of lymphocytes, epithelioid cells, foamy histiocytes and giant cells, many of them of Touton type. Some patients who had lesions are asymptomatic, sometimes they will feel paresthesias, burning pain. Nowadays, this management still vary widely, include medicamentous (cytostatics, steroids), radiotherapy, and surgery. Sets forth the results of two patients NXG. Methods: The subjects in this study were a 44-years-old male patient with some lesions on both cheeks and forehead since 5 months ago and a 29-years-old female patient with some lesions on both cheeks and ears. Results: First patient treated with Methylprednisolon 0.8 mg/Kg and tappered off for a month with improved results. Second patient treated with Cyclosphosphamide 750 mg/m2 with improved results within three weeks. Conclusion: Necrobiotic Xanthogranuloma treatment still required further research by the number of samples that much to find out the efficiency management NXG. Keywords: Cyclosphosphamide, methylprednisolon, necrobiotic xanthogranuloma 1 Introduction Necrobiotic Xanthogranuloma (NXG) is a rare disease, a chronic, progressive and cause skin lesions in the form of damage to the cell Non-Lagerhans histiocytes. -
An Unusual Juvenile Xanthogranuloma on a Finger MCP Joint
200 An Unusual Juvenile Xanthogranuloma on a Finger MCP Joint Sang Hee Cha, M.D., Sang Hyun Cho, M.D., Jeong Deuk Lee, M.D. Department of Dermatology, College of Medicine, The Catholic University of Korea, Seoul, Korea Juvenile xanthogranuloma (JXG) is a benign self-limited histiocytic proliferative disorder that usually occurs in early childhood. JXG appears as reddish to yellow, papules, or nodules, and although the head, neck, and trunk are the most frequent locations, it can occur at any body site. However, JXG involving the finger is rare. Histologically, JXG is characterized by an ill-defined, unencapsulated, dense histiocytic infiltrate within the dermis, some of which is contained in Touton giant cells, foreign body giant cells and foamy cells. Because the cutaneous lesions spontaneously regress, treatment is not usually indicated. The authors report a case of JXG in a 4-year-old girl who had tender, yellowish papule on the ventral aspect of the MCP joint of the right fourth finger consistent with JXG. (Ann Dermatol (Seoul) 20(4) 200∼203, 2008) Key Words: Finger, Juvenile xanthogranuloma INTRODUCTION CASE REPORT Juvenile xanthogranuloma (JXG) is a benign A 4-year-old girl presented with a papule of cutaneous histiocytic proliferation, and was first several months duration on the ventral aspect of described by Helwig and Hackney in 19541. The the right fourth finger MCP joint (Fig. 1). The pathophysiology of JXG is not well understood, lesion was a firm, dome-shaped, yellowish, 0.4×0.4 although it is thought to originate from a histiocytic cm sized papule. There was no remarkable past or granulomatous reaction2,3. -
Supplementary Table 1: Adhesion Genes Data Set
Supplementary Table 1: Adhesion genes data set PROBE Entrez Gene ID Celera Gene ID Gene_Symbol Gene_Name 160832 1 hCG201364.3 A1BG alpha-1-B glycoprotein 223658 1 hCG201364.3 A1BG alpha-1-B glycoprotein 212988 102 hCG40040.3 ADAM10 ADAM metallopeptidase domain 10 133411 4185 hCG28232.2 ADAM11 ADAM metallopeptidase domain 11 110695 8038 hCG40937.4 ADAM12 ADAM metallopeptidase domain 12 (meltrin alpha) 195222 8038 hCG40937.4 ADAM12 ADAM metallopeptidase domain 12 (meltrin alpha) 165344 8751 hCG20021.3 ADAM15 ADAM metallopeptidase domain 15 (metargidin) 189065 6868 null ADAM17 ADAM metallopeptidase domain 17 (tumor necrosis factor, alpha, converting enzyme) 108119 8728 hCG15398.4 ADAM19 ADAM metallopeptidase domain 19 (meltrin beta) 117763 8748 hCG20675.3 ADAM20 ADAM metallopeptidase domain 20 126448 8747 hCG1785634.2 ADAM21 ADAM metallopeptidase domain 21 208981 8747 hCG1785634.2|hCG2042897 ADAM21 ADAM metallopeptidase domain 21 180903 53616 hCG17212.4 ADAM22 ADAM metallopeptidase domain 22 177272 8745 hCG1811623.1 ADAM23 ADAM metallopeptidase domain 23 102384 10863 hCG1818505.1 ADAM28 ADAM metallopeptidase domain 28 119968 11086 hCG1786734.2 ADAM29 ADAM metallopeptidase domain 29 205542 11085 hCG1997196.1 ADAM30 ADAM metallopeptidase domain 30 148417 80332 hCG39255.4 ADAM33 ADAM metallopeptidase domain 33 140492 8756 hCG1789002.2 ADAM7 ADAM metallopeptidase domain 7 122603 101 hCG1816947.1 ADAM8 ADAM metallopeptidase domain 8 183965 8754 hCG1996391 ADAM9 ADAM metallopeptidase domain 9 (meltrin gamma) 129974 27299 hCG15447.3 ADAMDEC1 ADAM-like, -
A New Insight Into Viral Proteins As Immunomodulatory Therapeutic Agents
Iranian Journal of Basic Medical Sciences ijbms.mums.ac.ir A new insight into viral proteins as Immunomodulatory therapeutic agents. KSHV vOX2 a homolog of human CD200 as a potent anti-inflammatory protein Maryam Mousavinezhad-Moghaddam 1, Abbas Ali Amin 2, Houshang Rafatpanah 3, Seyed Abdol Rahim Rezaee 4 * 1 Department of Physiology, Biology Division, Faculty of Sciences, Ferdowsi University of Mashhad, Mashhad, Iran 2 Department of Immunology, Faculty of Medicine, Kurdistan University of Medical Sciences, Sanandaj, Iran 3 Immunology Research Centre, Bu-Ali Research Institute, Mashhad University of Medical Sciences, Mashhad, Iran 4 Inflammation and Inflammatory Diseases Research Center, Faculty of Medicine, Mashhad University of Medical Sciences, Mashhad, Iran A R T I C L E I N F O A B S T R A C T Article type: Review article The physiologic function of the immune system is defence against infectious microbes and tumour Article history: cells, Therefore, need to have precise modulatory mechanisms to maintain the body homeostasis. The Received: Aug 12, 2014 mammalian cellular CD200 (OX2)/CD200R interaction is one of such modulatory mechanisms in which Accepted: Nov 13, 2014 myeloid and lymphoid cells are regulated. CD200 and CD200R molecules are membrane proteins that Keywords: their immunomodulatory effects are able to suppress inflammatory responses, particularly in the CD200 privilege sites such as CNS and eyes. Kaposi’s sarcoma-associated herpesvirus (KSHV), encodes a wide Immune modulation variety of immunoregulatory proteins which play central roles in modulating inflammatory and anti- KSHV inflammatory responses in favour of virus dissemination. One such protein is a homologue of the RGD human CD200, encoded by open reading frame (ORF) K14 and therefore called vOX2/vCD200. -
Review Article the Modulatory Effects of Mesenchymal Stem Cells on Osteoclastogenesis
Hindawi Publishing Corporation Stem Cells International Volume 2016, Article ID 1908365, 13 pages http://dx.doi.org/10.1155/2016/1908365 Review Article The Modulatory Effects of Mesenchymal Stem Cells on Osteoclastogenesis Wessam E. Sharaf-Eldin,1,2 Nourhan Abu-Shahba,2,3 Marwa Mahmoud,1,2,3 and Nagwa El-Badri1 1 Center of Excellence of Stem Cells and Regenerative Medicine, Zewail City of Science and Technology, Sheikh Zayed District, 6thofOctoberCity,Giza12566,Egypt 2Medical Molecular Genetics Department, Human Genetics and Genome Research Division, National Research Centre, Cairo 12411, Egypt 3Stem Cells Research Group, Centre of Excellence for Advanced Sciences, National Research Centre, Cairo 12411, Egypt Correspondence should be addressed to Nagwa El-Badri; [email protected] Received 12 July 2015; Accepted 21 September 2015 AcademicEditor:JoelC.Glover Copyright © 2016 Wessam E. Sharaf-Eldin et al. This is an open access article distributed under the Creative Commons Attribution License, which permits unrestricted use, distribution, and reproduction in any medium, provided the original work is properly cited. The effect of mesenchymal stem cells (MSCs) on bone formation has been extensively demonstrated through several in vitro and in vivo studies. However, few studies addressed the effect of MSCs on osteoclastogenesis and bone resorption. Under physiological conditions, MSCs support osteoclastogenesis through producing the main osteoclastogenic cytokines, RANKL and M-CSF. However, during inflammation, MSCs suppress osteoclast formation and activity, partly via secretion of the key anti- osteoclastogenic factor, osteoprotegerin (OPG). In vitro, co-culture of MSCs with osteoclasts in the presence of high concentrations of osteoclast-inducing factors might reflect the in vivo inflammatory pathology and prompt MSCs to exert an osteoclastogenic suppressive effect. -
Molecular Analyses of Malignant Pleural Mesothelioma
Molecular Analyses of Malignant Pleural Mesothelioma Shir Kiong Lo National Heart and Lung Institute Imperial College Dovehouse Street London SW3 6LY A thesis submitted for MD (Res) Faculty of Medicine, Imperial College London 2016 1 Abstract Malignant pleural mesothelioma (MPM) is an aggressive cancer that is strongly associated with asbestos exposure. Majority of patients with MPM present with advanced disease and the treatment paradigm mainly involves palliative chemotherapy and best supportive care. The current chemotherapy options are limited and ineffective hence there is an urgent need to improve patient outcomes. This requires better understanding of the genetic alterations driving MPM to improve diagnostic, prognostic and therapeutic strategies. This research aims to gain further insights in the pathogenesis of MPM by exploring the tumour transcriptional and mutational profiles. We compared gene expression profiles of 25 MPM tumours and 5 non-malignant pleura. This revealed differentially expressed genes involved in cell migration, invasion, cell cycle and the immune system that contribute to the malignant phenotype of MPM. We then constructed MPM-associated co-expression networks using weighted gene correlation network analysis to identify clusters of highly correlated genes. These identified three distinct molecular subtypes of MPM associated with genes involved in WNT and TGF-ß signalling pathways. Our results also revealed genes involved in cell cycle control especially the mitotic phase correlated significantly with poor prognosis. Through exome analysis of seven paired tumour/blood and 29 tumour samples, we identified frequent mutations in BAP1 and NF2. Additionally, the mutational profile of MPM is enriched with genes encoding FAK, MAPK and WNT signalling pathways. -
CD200 in Hematological Malignancies: Just a Diagnostic Tool Or More?
Editorial Page 1 of 4 CD200 in hematological malignancies: just a diagnostic tool or more? Daniela Damiani, Mario Tiribelli Division of Hematology and Stem Cell Transplantation, Department of Medical Area, Azienda Sanitaria Universitaria Integrata di Udine, Udine, Italy Correspondence to: Prof. Daniela Damiani. Division of Hematology and Stem Cell Transplantation Department of Medical Area, Azienda Sanitaria Universitaria Integrata di Udine, 33100 Udine, Italy. Email: [email protected]. Provenance: This is a Guest Editorial commissioned by the Section Editor Baohong Yue (Hematology lab in Department of Clinical Laboratory Medicine, The First Affiliated Hospital, College of Medicine, Zhengzhou University, Zhengzhou, China). Comment on: Sandes AF, de Lourdes Chauffaille M, Oliveira CR, et al. CD200 has an important role in the differential diagnosis of mature B cell neoplasms by multiparameter flow cytometry. Cytometry B Clin Cytom 2014;86:98-105. Received: 28 July 2017; Accepted: 08 August 2017; Published: 15 September 2017. doi: 10.21037/jlpm.2017.08.08 View this article at: http://dx.doi.org/10.21037/jlpm.2017.08.08 Over the past two decades, the increased number of monoclonal Cytometry, Sandes and colleagues tested the diagnostic antibodies and the constantly expanding availability of utility of CD200 in differential diagnosis of 159 patients fluorescence probes significantly improved the efficiency with mature B lymphoproliferative diseases (3). They and the accuracy of flow cytometric analysis, providing not only added CD200 to a conventional MFC panel, relevant information for the diagnosis, classification and evaluating its dichotomous expression, but also compared follow up of various hematological malignancies. As a the fluorescence intensity in the tested cases, with the aim to diagnostic tool, together with morphology and molecular create a reference pool of CD200 expression intensity that genetics, flow cytometry has become a gold standard in may further discriminate within the CD200-positive group.