In Silico Prediction and Analysis of Unusual Lantibiotic Resistance Operons in the Genus Corynebacterium
Total Page:16
File Type:pdf, Size:1020Kb
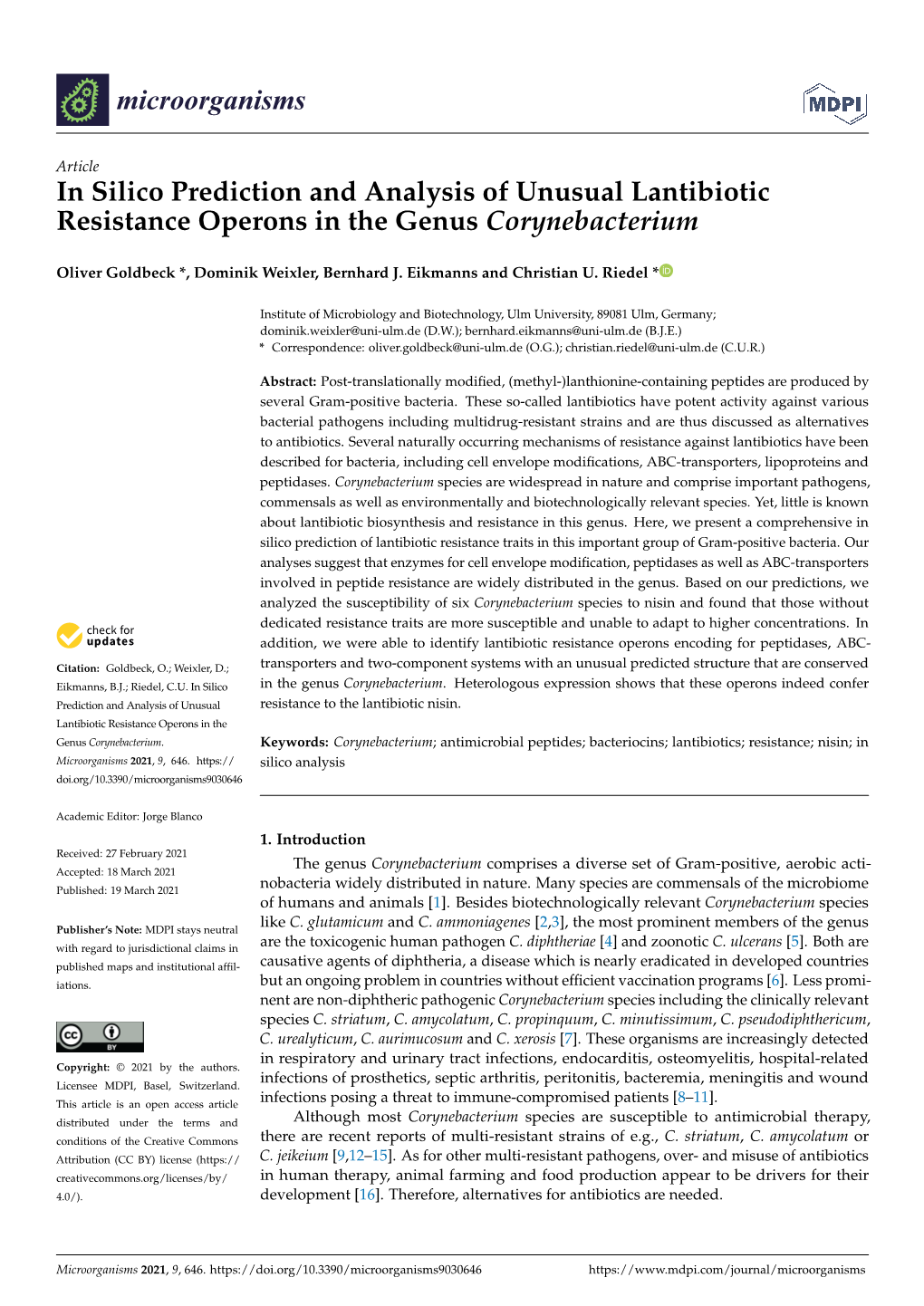
Load more
Recommended publications
-
NISIN: PRODUCTION and MECHANISM of ANTIMICROBIAL ACTION IJCRR Section: Healthcare Sci
Review Article NISIN: PRODUCTION AND MECHANISM OF ANTIMICROBIAL ACTION IJCRR Section: Healthcare Sci. Journal Impact Factor Sukrita Punyauppa-path, Parichat Phumkhachorn, 4.016 Pongsak Rattanachaikunsopon Department of Biological Science, Faculty of Science, Ubon Ratchathani University, Ubon Ratchathani 34190, Thailand. ABSTRACT Nisin is a heat stable lantibiotic consisting of 34 amino acids. Of these amino acids, there are several unusual amino acids includ- ing dehydroalanine, dehydrobutyrine, aminobutyric acid, lanthionine and β-methyllanthionine. It has antimicrobial activity against many species of Gram positive bacteria, but not Gram negative bacteria due to their outer membrane barriers. However, when used in combination with other chemical or physical treatments that destabilize the outer membranes, nisin can inhibit Gram negative bacteria. Nisin has been used as a food preservative in many food industries because it is legally approved as safe for use in food and beverage. The knowledge on the production and mechanism of antimicrobial action of nisin is important for the understanding how nisin contains unusual amino acids and how it kills sensitive bacteria. The knowledge may also be a factor for the successful application of nisin. Therefore, this review focuses on presenting these two aspects of nisin. Key Words: Bacteriocin, Lactococcus lactis, lanbiotic, nisin INTRODUCTION (Table 1), but not Gram negative bacteria due to their outer membrane barrier. Normally, nisin producer has Nisin is the antimcirobial peptide produced by Lactococ- immunity to its own produced nisin but not to other lan- 1 cus lactis subsp. Lactis . It is the only bacterioicin that tibotics. This is for protecting itself from being killed by have been legally approved as safe for use in food and its own nisin. -
Bacteriotherapy in Breast Cancer
International Journal of Molecular Sciences Review Bacteriotherapy in Breast Cancer 1,2, 3, 4 5 Atieh Yaghoubi y, Majid Khazaei y, Seyed Mahdi Hasanian , Amir Avan , William C. Cho 6,* and Saman Soleimanpour 1,2,* 1 Antimicrobial Resistance Research Center, Bu-Ali Research Institute, Mashhad University of Medical Sciences, Mashhad 91387-35499, Iran; [email protected] 2 Department of Microbiology and Virology, Faculty of Medicine, Mashhad University of Medical Sciences, Mashhad 91387-35499, Iran 3 Department of Physiology, Faculty of Medicine, Mashhad University of Medical Sciences, Mashhad 9138735499, Iran; [email protected] 4 Department of Medical Biochemistry, Faculty of Medicine, Mashhad University of Medical, Sciences, Mashhad 91387-35499, Iran; [email protected] 5 Cancer Research Center, Mashhad University of Medical Sciences, Mashhad 91387-35499, Iran; [email protected] 6 Department of Clinical Oncology, Queen Elizabeth Hospital, Kowloon, Hong Kong * Correspondence: [email protected] or [email protected] (W.C.C.); [email protected] (S.S.); Tel.: +852-3506-6284 (W.C.C.); +98-912-6590-092 (S.S.); Fax: +852-3506-5455 (W.C.C.); +98-511-8409-612 (S.S.) These authors contributed equally to this work. y Received: 2 October 2019; Accepted: 18 November 2019; Published: 23 November 2019 Abstract: Breast cancer is the second most common cause of cancer-related mortality among women around the world. Conventional treatments in the fight against breast cancer, such as chemotherapy, are being challenged regarding their effectiveness. Thus, strategies for the treatment of breast cancer need to be continuously refined to achieve a better patient outcome. -
First Evidence of Production of the Lantibiotic Nisin P Enriqueta Garcia-Gutierrez1,2, Paula M
www.nature.com/scientificreports OPEN First evidence of production of the lantibiotic nisin P Enriqueta Garcia-Gutierrez1,2, Paula M. O’Connor2,3, Gerhard Saalbach4, Calum J. Walsh2,3, James W. Hegarty2,3, Caitriona M. Guinane2,5, Melinda J. Mayer1, Arjan Narbad1* & Paul D. Cotter2,3 Nisin P is a natural nisin variant, the genetic determinants for which were previously identifed in the genomes of two Streptococcus species, albeit with no confrmed evidence of production. Here we describe Streptococcus agalactiae DPC7040, a human faecal isolate, which exhibits antimicrobial activity against a panel of gut and food isolates by virtue of producing nisin P. Nisin P was purifed, and its predicted structure was confrmed by nanoLC-MS/MS, with both the fully modifed peptide and a variant without rings B and E being identifed. Additionally, we compared its spectrum of inhibition and minimum inhibitory concentration (MIC) with that of nisin A and its antimicrobial efect in a faecal fermentation in comparison with nisin A and H. We found that its antimicrobial activity was less potent than nisin A and H, and we propose a link between this reduced activity and the peptide structure. Nisin is a small peptide with antimicrobial activity against a wide range of pathogenic bacteria. It was originally sourced from a Lactococcus lactis subsp. lactis isolated from a dairy product1 and is classifed as a class I bacteri- ocin, as it is ribosomally synthesised and post-translationally modifed2. Nisin has been studied extensively and has a wide range of applications in the food industry, biomedicine, veterinary and research felds3–6. -
Functional Interaction of Human Neutrophil Peptide-1 with the Cell Wall Precursor Lipid II
View metadata, citation and similar papers at core.ac.uk brought to you by CORE provided by Elsevier - Publisher Connector FEBS Letters 584 (2010) 1543–1548 journal homepage: www.FEBSLetters.org Functional interaction of human neutrophil peptide-1 with the cell wall precursor lipid II Erik de Leeuw a,*, Changqing Li a, Pengyun Zeng b, Chong Li b, Marlies Diepeveen-de Buin c, Wei-Yue Lu b, Eefjan Breukink c, Wuyuan Lu a,** a University of Maryland Baltimore School of Medicine, Institute of Human Virology and Department of Biochemistry and Molecular Biology, 725 West Lombard Street, Baltimore, MD 21201, USA b Fudan University School of Pharmacy, Shanghai, China c Utrecht University, Department of Biochemistry of Membranes, Bijvoet Center for Biomolecular Research, Padualaan 8, 3585 CH, Utrecht, The Netherlands article info abstract Article history: Defensins constitute a major class of cationic antimicrobial peptides in mammals and vertebrates, Received 11 January 2010 acting as effectors of innate immunity against infectious microorganisms. It is generally accepted Revised 1 March 2010 that defensins are bactericidal by disrupting the anionic microbial membrane. Here, we provide evi- Accepted 2 March 2010 dence that membrane activity of human a-defensins does not correlate with antibacterial killing. Available online 7 March 2010 We further show that the a-defensin human neutrophil peptide-1 (HNP1) binds to the cell wall pre- Edited by Renee Tsolis cursor lipid II and that reduction of lipid II levels in the bacterial membrane significantly reduces bacterial killing. The interaction between defensins and lipid II suggests the inhibition of cell wall synthesis as a novel antibacterial mechanism of this important class of host defense peptides. -
UC San Diego UC San Diego Electronic Theses and Dissertations
UC San Diego UC San Diego Electronic Theses and Dissertations Title Probiogenomic Analysis of Three Commonly Occuring Bifidobacterial Species Permalink https://escholarship.org/uc/item/8d67d715 Author Kim, Andrew Min Publication Date 2018 Peer reviewed|Thesis/dissertation eScholarship.org Powered by the California Digital Library University of California UNIVERSITY OF CALIFORNIA SAN DIEGO Probiogenomic Analysis of Three Commonly Occuring Bifidobacterial Species A Thesis submitted in partial satisfaction of the requirements for the degree Master of Science in Biology by Andrew Min Kim Committee in charge: Milton Saier, Chair Eric Allen, Co-Chair Stanley Lo 2018 © Copyright Andrew Min Kim, 2018 All rights reserved. The Thesis of Andrew Min Kim is approved, and it is acceptable in quality and form for publication on microfilm and electronically: Co-Chair Chair University of California San Diego 2018 iii TABLE OF CONTENTS Signature Page ........................................................................................................... iii Table of Contents ....................................................................................................... iv List of Tables ............................................................................................................. v Acknowledgements .................................................................................................... vi Abstract of the Thesis ................................................................................................ vii Introduction -
Alternative Functions of ABC Transporters in Bacillus Subtilis and Listeria Monocytogenes
microorganisms Review Not Just Transporters: Alternative Functions of ABC Transporters in Bacillus subtilis and Listeria monocytogenes Jeanine Rismondo * and Lisa Maria Schulz Department of General Microbiology, GZMB, Georg-August-University Göttingen, Grisebachstr. 8, D-37077 Göttingen, Germany; [email protected] * Correspondence: [email protected]; Tel.: +49-551-39-33796 Abstract: ATP-binding cassette (ABC) transporters are usually involved in the translocation of their cognate substrates, which is driven by ATP hydrolysis. Typically, these transporters are required for the import or export of a wide range of substrates such as sugars, ions and complex organic molecules. ABC exporters can also be involved in the export of toxic compounds such as antibiotics. However, recent studies revealed alternative detoxification mechanisms of ABC transporters. For instance, the ABC transporter BceAB of Bacillus subtilis seems to confer resistance to bacitracin via target protection. In addition, several transporters with functions other than substrate export or import have been identified in the past. Here, we provide an overview of recent findings on ABC transporters of the Gram-positive organisms B. subtilis and Listeria monocytogenes with transport or regulatory functions affecting antibiotic resistance, cell wall biosynthesis, cell division and sporulation. Keywords: ABC transporter; antibiotic resistance; Gram-positive bacteria; cell wall 1. Introduction ATP-binding cassette (ABC) transporters can be found in all kingdoms of life and Citation: Rismondo, J.; Schulz, L.M. can be subdivided in three main groups: eukaryotic transporters, bacterial importers and Not Just Transporters: Alternative bacterial exporters. In these transporters, the translocation of cognate substrates is driven Functions of ABC Transporters in by ATP hydrolysis [1]. -
Lantibiotics Produced by Oral Inhabitants As a Trigger for Dysbiosis of Human Intestinal Microbiota
International Journal of Molecular Sciences Article Lantibiotics Produced by Oral Inhabitants as a Trigger for Dysbiosis of Human Intestinal Microbiota Hideo Yonezawa 1,* , Mizuho Motegi 2, Atsushi Oishi 2, Fuhito Hojo 3 , Seiya Higashi 4, Eriko Nozaki 5, Kentaro Oka 4, Motomichi Takahashi 1,4, Takako Osaki 1 and Shigeru Kamiya 1 1 Department of Infectious Diseases, Kyorin University School of Medicine, Tokyo 181-8611, Japan; [email protected] (M.T.); [email protected] (T.O.); [email protected] (S.K.) 2 Division of Oral Restitution, Department of Pediatric Dentistry, Graduate School, Tokyo Medical and Dental University, Tokyo 113-8510, Japan; [email protected] (M.M.); [email protected] (A.O.) 3 Institute of Laboratory Animals, Graduate School of Medicine, Kyorin University School of Medicine, Tokyo 181-8611, Japan; [email protected] 4 Central Research Institute, Miyarisan Pharmaceutical Co. Ltd., Tokyo 114-0016, Japan; [email protected] (S.H.); [email protected] (K.O.) 5 Core Laboratory for Proteomics and Genomics, Kyorin University School of Medicine, Tokyo 181-8611, Japan; [email protected] * Correspondence: [email protected] Abstract: Lantibiotics are a type of bacteriocin produced by Gram-positive bacteria and have a wide spectrum of Gram-positive antimicrobial activity. In this study, we determined that Mutacin I/III and Smb (a dipeptide lantibiotic), which are mainly produced by the widespread cariogenic bacterium Streptococcus mutans, have strong antimicrobial activities against many of the Gram-positive bacteria which constitute the intestinal microbiota. -
Structural and Functional Characterization of the Lantibiotic Mutacin 1140
STRUCTURAL AND FUNCTIONAL CHARACTERIZATION OF THE LANTIBIOTIC MUTACIN 1140 By JAMES LEIF SMITH A DISSERTATION PRESENTED TO THE GRADUATE SCHOOL OF THE UNIVERSITY OF FLORIDA IN PARTIAL FULFILLMENT OF THE REQUIREMENTS FOR THE DEGREE OF DOCTOR OF PHILOSOPHY UNIVERSITY OF FLORIDA 2002 Copyright 2002 by James Leif Smith This work is dedicated to all my family and friends. Finishing this Ph.D. has come down to two traits that my family instilled in me. One is to never give up, and the other is the lack of good common sense. My friends for standing by my side while I complained moaned and groaned about my life over the last five years. ACKNOWLEDGMENTS First and foremost, I am thankful to my parents for all the love and guidance they have given me throughout my life. I would like to thank my lab mates Cherian Zachariah, Eduardo Espinoza, Matt Carrigan, Ramanan Thirumoorthy, Steve Thomas, Terry Green, and Alexis Harrison for their help and most of all for their friendship. I would like to thank my mentor Arthur S. Edison for teaching me patience. I am very thankful to him for all that he has done for me and for his patience with me. I have learned very much in the time I spent in his lab. I would like to thank my committee members Nancy Denslow, Ben Dunn, Jeff Hillman, and Gerry Shaw for all their help with my project. In particular I would like to thank the Department of Oral Biology and Jeffrey Hillman for supporting my work. I would also like to thank all of my committee members for supporting my decision to enter the MBA program. -
Antibacterial Effect of High-Purity Nisin Alone and in Combination with D-Amino Acids Or Chlorhexidine in an Endodontic-Like Biofilm Model
antibiotics Article Antibacterial Effect of High-Purity Nisin Alone and in Combination with D-Amino Acids or Chlorhexidine in an Endodontic-Like Biofilm Model Ericka T. Pinheiro 1,2,* , Lamprini Karygianni 2 , Thomas Attin 2 and Thomas Thurnheer 2 1 Department of Dentistry, School of Dentistry, University of São Paulo, São Paulo 01000-000, Brazil 2 Clinic of Conservative and Preventive Dentistry, Center of Dental Medicine, University of Zurich, CH-8032 Zürich, Switzerland; [email protected] (L.K.); [email protected] (T.A.); [email protected] (T.T.) * Correspondence: [email protected] or [email protected]; Tel.: +41-44-634-32-56 Abstract: New strategies to eradicate endodontic biofilms are needed. Therefore, we evaluated the effect of high-purity nisin alone and in combination with D-amino acids (D-AAs) or chlorhexidine (CHX) against an “endodontic-like” biofilm model. Biofilms were grown on hydroxyapatite discs for 64 h and treated with nisin, eight D-AAs mixture, nisin + eight D-AAs, 2% CHX, and nisin + 2% CHX. After the 5 min and 24 h treatments, biofilm cells were harvested and total colony-forming units were counted. Differences between groups were tested by two-way ANOVA followed by Tukey’s multiple comparisons test (p < 0.05). Nisin and D-AAs, alone or in combination, were not effective in reducing bacteria after short or long exposure times. After 5 min, treatment with 2% CHX and nisin + 2% CHX resulted in 2 and 2.4-log cell reduction, respectively, compared with the no treatment control (p < 0.001). -
NISIN and the MARKET for COMMERICIAL BACTERIOCINS Dr
NISIN AND THE MARKET FOR COMMERICIAL BACTERIOCINS Dr. Eluned Jones Dr. Victoria Salin Dr. Gary W. Williams* TAMRC Consumer and Product Research Report No. CP-01-05 July 2005 * Jones and Salin are Associate Professors and Williams is Professor of Agricultural Economics and Director, Texas Agricultural Market Research, Department of Agricultural Economics, Texas A&M University, College Station, Texas 77843-2124. NISIN AND THE MARKET FOR COMMERICIAL BACTERIOCINS Texas Agribusiness Market Research Center (TAMRC) Consumer and Product Research Report No. CP-01-05 by Dr. Eluned Jones, Dr. Victoria Salin, and Dr. Gary W. Williams, July 2005. Abstract: This report provides a background analysis of the market for nisin and other commercially available antimicrobial food additives. The report concludes that there is a potential role for a new U.S.-based entity to compete with a nisin product that is cost-competitive or provides quality guarantees to satisfy U.S. buyers who have tight specifications for ingredient sourcing and food safety and quality oversight. Acknowledgements The research reported here was conducted under contract for the National Corn Growers Association (NCGA). Thanks are due to Richard Glass and Nathan Fields of the NCGA for their support. The conclusions reached and any views expressed, however, are those of the authors and may not represent those of NCGA or of Texas A&M University. The Texas Agribusiness Market Research Center (TAMRC) has been providing timely, unique, and professional research on a wide range of issues relating to agricultural and agribusiness markets and products of importance to Texas and the nation for thirty-five years. -
Thelactococcus Lactis Nisin-Sucrose Conjugative Transposon Tti5276
The Lactococcus lactis Nisin-Sucrose Conjugative Transposon Tti5276 0000 0513475 0 [: ijC, $ Promoter: dr. W. M. deVo s hoogleraar in de bacteriele genetica 3 fjrj 02?€>\ ^ f Peter Jacobus GerardusRauc h The Lactococcus lactisNisin-Sucros e Conjugative Transposon Tn5276 Proefschrift ter verkrijging van de graad van doctor in de landbouw- enmilieuwetenschappe n op gezag van de rector magnificus dr. H. C. van der Plas, in het openbaar te verdedigen op woensdag 26 mei 1993 des namiddags te vier uur in de Aula van de Landbouwuniversiteit te Wageningen \^v \ ; ^ "A% -^ c\ \ J De natuur is beweging. James Hutton Tendage dat ikriep, hebtGij mi) geantwoord, Gijhebt mi) bemoedigd metkracht in mijn ziel. Psalm 138(NB G vertaling) BIBLIOTHEER OSNDBOUWUNIVEBSOBW SPAGENlNGEtt Aan papen mam, Catelijne, Marijke en Lonneke ] I-JI y)3X> i STELLINGEN 1. Debewerin gva nPoyart-Salmero n etal. datd eplaats-specifiek e recombinatie-reactie van Tnl545 zich onderscheidt van die van bacteriofaag Xdoorda t het Int-Tn eiwit ook functioneert in afwezigheid van Xis-Tn is onjuist. Poyart-Salmeron, C, P. Trieu-Cuot, C. Carlier en P. Courvalin. 1989. Molecular characterization of twoprotein s involved inth e excision ofth e conjugative transposon Tnl545: homologies with other site-specific recombinases. EMBOJ . 8:2425-2433. Abremski, K. enS. Gottesman. 1981. Site-specific recombination. Xis-independent excisive recombination of bacteriophage lambda. J. Mol. Biol. 153:67-78. De bewering van Steen et al. dat het structurele nisine gen van Lactococcus lactis ATCC 11454 afgeschreven wordt vanuit een promoter die meer dan 4 kb stroomopwaarts ligt, is onwaarschijnlijk aangezien transcriptie van dit gen dan afhankelijk zou zijn van de plaats van insertie van het nisine-sucrose element in het chromosoom en van mogelijke IS-gemedieerde herrangschikkingen van het gebied tussen het startpunt van transcriptie en het nisine gen. -
Characterisation of the ATP-Binding Cassette Transporters Of
Characterisation Of The ATP-Binding Cassette Transporters Of Pseudomonas aeruginosa Victoria Grace Pederick Research Centre for Infectious Diseases, Department of Microbiology and Immunology, School of Molecular and Biomedical Sciences, University of Adelaide October 2014 TABLE OF CONTENTS ABSTRACT ............................................................................................................................... V DECLARATION .................................................................................................................... VII COPYRIGHT STATEMENT ................................................................................................ VIII ABBREVIATIONS ................................................................................................................. IX TABLE OF TABLES ............................................................................................................. XII TABLE OF FIGURES ........................................................................................................... XIII ACKNOWLEDGEMENTS .................................................................................................... XV CHAPTER 1: INTRODUCTION ........................................................................................ 1 Pseudomonas aeruginosa ........................................................................................... 1 1.1.1. P. aeruginosa and human disease ......................................................................... 1 1.1.1.1. Cystic