Zooplankton Community Structure in a Cyclonic and Mode-Water Eddy in the Sargasso Sea
Total Page:16
File Type:pdf, Size:1020Kb
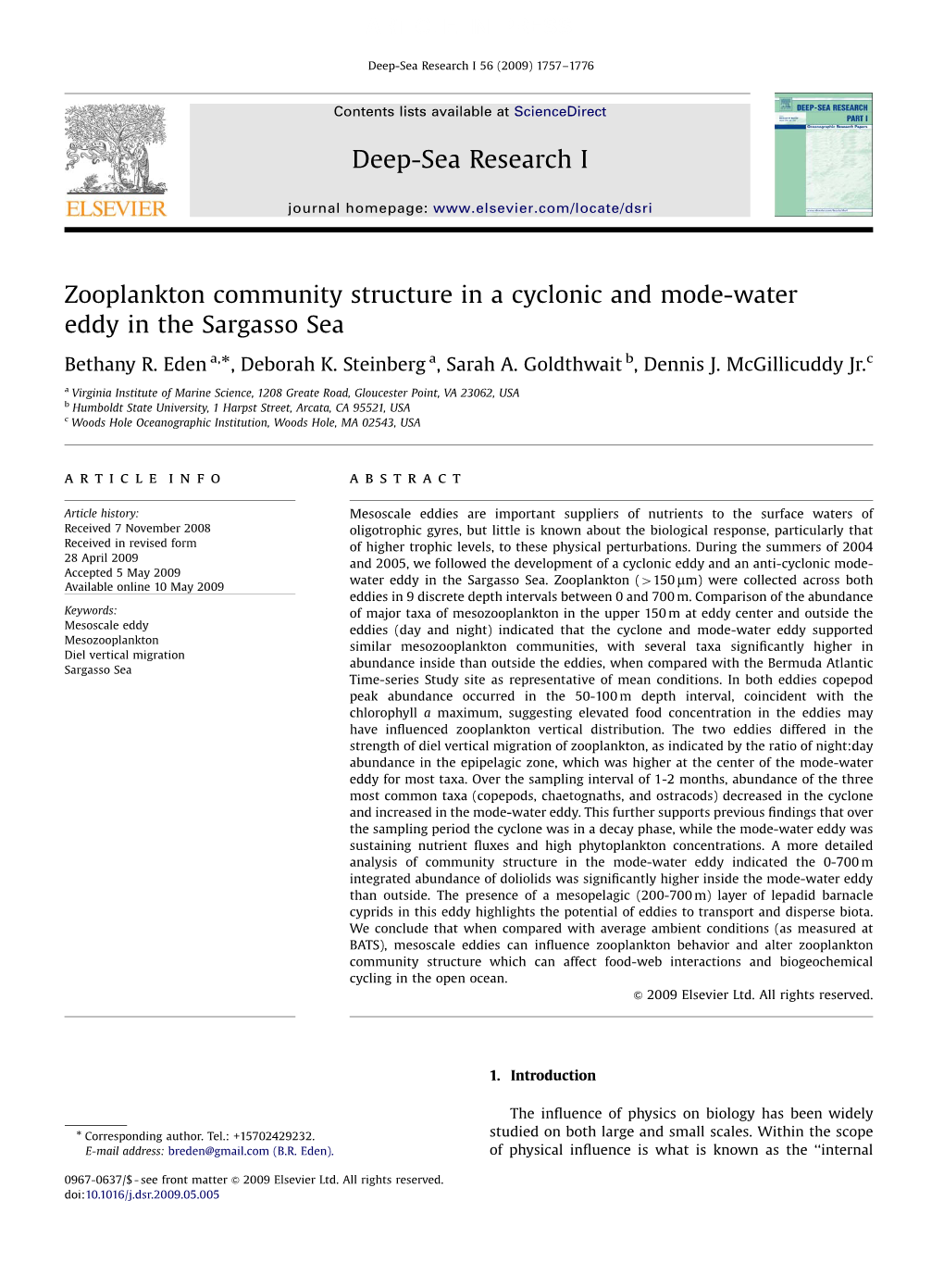
Load more
Recommended publications
-
Understanding Eddy Field in the Arctic Ocean from High-Resolution Satellite Observations Igor Kozlov1, A
Abstract ID: 21849 Understanding eddy field in the Arctic Ocean from high-resolution satellite observations Igor Kozlov1, A. Artamonova1, L. Petrenko1, E. Plotnikov1, G. Manucharyan2, A. Kubryakov1 1Marine Hydrophysical Institute of RAS, Russia; 2School of Oceanography, University of Washington, USA Highlights: - Eddies are ubiquitous in the Arctic Ocean even in the presence of sea ice; - Eddies range in size between 0.5 and 100 km and their orbital velocities can reach up to 0.75 m/s. - High-resolution SAR data resolve complex MIZ dynamics down to submesoscales [O(1 km)]. Fig 1. Eddy detection Fig 2. Spatial statistics Fig 3. Dynamics EGU2020 OS1.11: Changes in the Arctic Ocean, sea ice and subarctic seas systems: Observations, Models and Perspectives 1 Abstract ID: 21849 Motivation • The Arctic Ocean is a host to major ocean circulation systems, many of which generate eddies transporting water masses and tracers over long distances from their formation sites. • Comprehensive observations of eddy characteristics are currently not available and are limited to spatially and temporally sparse in situ observations. • Relatively small Rossby radii of just 2-10 km in the Arctic Ocean (Nurser and Bacon, 2014) also mean that most of the state-of-art hydrodynamic models are not eddy-resolving • The aim of this study is therefore to fill existing gaps in eddy observations in the Arctic Ocean. • To address it, we use high-resolution spaceborne SAR measurements to detect eddies over the ice-free regions and in the marginal ice zones (MIZ). EGU20 -OS1.11 – Kozlov et al., Understanding eddy field in the Arctic Ocean from high-resolution satellite observations 2 Abstract ID: 21849 Methods • We use multi-mission high-resolution spaceborne synthetic aperture radar (SAR) data to detect eddies over open ocean and marginal ice zones (MIZ) of Fram Strait and Beaufort Gyre regions. -
The General Circulation of the Atmosphere and Climate Change
12.812: THE GENERAL CIRCULATION OF THE ATMOSPHERE AND CLIMATE CHANGE Paul O'Gorman April 9, 2010 Contents 1 Introduction 7 1.1 Lorenz's view . 7 1.2 The general circulation: 1735 (Hadley) . 7 1.3 The general circulation: 1857 (Thompson) . 7 1.4 The general circulation: 1980-2001 (ERA40) . 7 1.5 The general circulation: recent trends (1980-2005) . 8 1.6 Course aim . 8 2 Some mathematical machinery 9 2.1 Transient and Stationary eddies . 9 2.2 The Dynamical Equations . 12 2.2.1 Coordinates . 12 2.2.2 Continuity equation . 12 2.2.3 Momentum equations (in p coordinates) . 13 2.2.4 Thermodynamic equation . 14 2.2.5 Water vapor . 14 1 Contents 3 Observed mean state of the atmosphere 15 3.1 Mass . 15 3.1.1 Geopotential height at 1000 hPa . 15 3.1.2 Zonal mean SLP . 17 3.1.3 Seasonal cycle of mass . 17 3.2 Thermal structure . 18 3.2.1 Insolation: daily-mean and TOA . 18 3.2.2 Surface air temperature . 18 3.2.3 Latitude-σ plots of temperature . 18 3.2.4 Potential temperature . 21 3.2.5 Static stability . 21 3.2.6 Effects of moisture . 24 3.2.7 Moist static stability . 24 3.2.8 Meridional temperature gradient . 25 3.2.9 Temperature variability . 26 3.2.10 Theories for the thermal structure . 26 3.3 Mean state of the circulation . 26 3.3.1 Surface winds and geopotential height . 27 3.3.2 Upper-level flow . 27 3.3.3 200 hPa u (CDC) . -
Physical Oceanography and Circulation in the Gulf of Mexico
Physical Oceanography and Circulation in the Gulf of Mexico Ruoying He Dept. of Marine, Earth & Atmospheric Sciences Ocean Carbon and Biogeochemistry Scoping Workshop on Terrestrial and Coastal Carbon Fluxes in the Gulf of Mexico St. Petersburg, FL May 6-8, 2008 Adopted from Oey et al. (2005) Adopted from Morey et al. (2005) Averaged field of wind stress for the GOM. [adapted from Gutierrez de Velasco and Winant, 1996] Surface wind Monthly variability Spring transition vs Fall Transition Adopted from Morey et al. (2005) Outline 1. General circulation in the GOM 1.1. The loop current and Eddy Shedding 1.2. Upstream conditions 1.3. Anticyclonic flow in the central and northwestern Gulf 1.4. Cyclonic flow in the Bay of Campeche 1.5. Deep circulation in the Gulf 2. Coastal circulation 2.1. Coastal Circulation in the Eastern Gulf 2.2. Coastal Circulation in the Northern Gulf 2.3. Coastal Circulation in the Western Gulf 1. General Circulation in the GOM 1.1. The Loop Current (LC) and Eddy Shedding Eddy LC Summary statistics for the Loop Current metrics computed from the January1, 1993 through July 1, 2004 altimetric time series Leben (2005) A compilation of the 31-yr Record (July 1973 – June 2004) of LC separation event. The separation intervals vary From a few weeks up to ~ 18 Months. Separation intervals tend to Cluster near 4.5-7 and 11.5, And 17-18.5 months, perhaps Suggesting the possibility of ~ a 6 month duration between each cluster. Leben (2005); Schmitz et al. (2005) Sturges and Leben (1999) The question of why the LC and the shedding process behave in such a semi-erratic manner is a bit of a mystery • Hurlburt and Thompson (1980) found erratic eddy shedding intervals in the lowest eddy viscosity run in a sequence of numerical experiment. -
Pressure-Gradient Forcing Methods for Large-Eddy Simulations of Flows in the Lower Atmospheric Boundary Layer
atmosphere Article Pressure-Gradient Forcing Methods for Large-Eddy Simulations of Flows in the Lower Atmospheric Boundary Layer François Pimont 1,* , Jean-Luc Dupuy 1 , Rodman R. Linn 2, Jeremy A. Sauer 3 and Domingo Muñoz-Esparza 3 1 Department of Forest, Grassland and Freshwater Ecology, Unité de Recherche des Forets Méditerranéennes (URFM), French National Institute for Agriculture, Food, and Environment (INRAE), CEDEX 9, 84914 Avignon, France; [email protected] 2 Earth and Environmental Sciences Division, Los Alamos National Lab, Los Alamos, NM 87545, USA; [email protected] 3 Research Applications Laboratory, National Center for Atmospheric Research, Boulder, CO 80301, USA; [email protected] (J.A.S.); [email protected] (D.M.-E.) * Correspondence: [email protected]; Tel.: +33-432-722-947 Received: 26 October 2020; Accepted: 9 December 2020; Published: 11 December 2020 Abstract: Turbulent flows over forest canopies have been successfully modeled using Large-Eddy Simulations (LES). Simulated winds result from the balance between a simplified pressure gradient forcing (e.g., a constant pressure-gradient or a canonical Ekman balance) and the dissipation of momentum, due to vegetation drag. Little attention has been paid to the impacts of these forcing methods on flow features, despite practical challenges and unrealistic features, such as establishing stationary velocity or streak locking. This study presents a technique for capturing the effects of a pressure-gradient force (PGF), associated with atmospheric patterns much larger than the computational domain for idealized simulations of near-surface phenomena. Four variants of this new PGF are compared to existing forcings, for turbulence statistics, spectra, and temporal averages of flow fields. -
Advances in Marine Ecosystem Dynamics from US GLOBEC: the Horizontal-Advection Bottom-Up Forcing Paradigm
Advances in Marine Ecosystem Dynamics from US GLOBEC: The Horizontal-Advection Bottom-up Forcing Paradigm Di Lorenzo, E., D. Mountain, H.P. Batchelder, N. Bond, and E.E. Hofmann. 2013. Advances in marine ecosystem dynamics from US GLOBEC: The horizontal-advection bottom-up forcing paradigm. Oceanography 26(4):22–33. doi:10.5670/oceanog.2013.73 10.5670/oceanog.2013.73 Oceanography Society Version of Record http://hdl.handle.net/1957/47486 http://cdss.library.oregonstate.edu/sa-termsofuse SPECIAL ISSUE ON US GLOBEC: UNDERSTANDING CLIMATE IMPACTS ON MARINE ECOSYSTEMS Advances in Marine Ecosystem Dynamics from US GLOBEC The Horizontal-Advection Bottom-up Forcing Paradigm BY EMANUELE DI LORENZO, DAVID MOUNTAIN, HAROLD P. BATCHELDER, NICHOLAS BOND, AND EILEEN E. HOFMANN Southern Ocean GLOBEC cruise. Photo credit: Daniel Costa 22 Oceanography | Vol. 26, No. 4 ABSTRACT. A primary focus of the US Global Ocean Ecosystem Dynamics and atmosphere, the annular modes (GLOBEC) program was to identify the mechanisms of ecosystem response to large- are to first order uncoupled from ocean scale climate forcing under the assumption that bottom-up forcing controls a large variability and are associated with the fraction of marine ecosystem variability. At the beginning of GLOBEC, the prevailing strengthening and weakening of the bottom-up forcing hypothesis was that climate-induced changes in vertical transport atmospheric polar vortices. Together, modulated nutrient supply and surface primary productivity, which in turn affected these modes explain about one-third of the lower trophic levels (e.g., zooplankton) and higher trophic levels (e.g., fish) the global atmospheric variation that is through the trophic cascade. -
SWASHES: a Compilation of Shallow Water Analytic Solutions For
SWASHES: a compilation of Shallow Water Analytic Solutions for Hydraulic and Environmental Studies O. Delestre∗,‡,,† C. Lucas‡, P.-A. Ksinant‡,§, F. Darboux§, C. Laguerre‡, T.N.T. Vo‡,¶, F. James‡ and S. Cordier‡ January 22, 2016 Abstract Numerous codes are being developed to solve Shallow Water equa- tions. Because there are used in hydraulic and environmental studies, their capability to simulate properly flow dynamics is critical to guaran- tee infrastructure and human safety. While validating these codes is an important issue, code validations are currently restricted because analytic solutions to the Shallow Water equations are rare and have been published on an individual basis over a period of more than five decades. This article aims at making analytic solutions to the Shallow Water equations easily available to code developers and users. It compiles a significant number of analytic solutions to the Shallow Water equations that are currently scat- tered through the literature of various scientific disciplines. The analytic solutions are described in a unified formalism to make a consistent set of test cases. These analytic solutions encompass a wide variety of flow conditions (supercritical, subcritical, shock, etc.), in 1 or 2 space dimen- sions, with or without rain and soil friction, for transitory flow or steady state. The corresponding source codes are made available to the commu- nity (http://www.univ-orleans.fr/mapmo/soft/SWASHES), so that users of Shallow Water-based models can easily find an adaptable benchmark library to validate their numerical methods. Keywords Shallow-Water equation; Analytic solutions; Benchmarking; Vali- dation of numerical methods; Steady-state flow; Transitory flow; Source terms arXiv:1110.0288v7 [math.NA] 21 Jan 2016 ∗Corresponding author: [email protected], presently at: Laboratoire de Math´ematiques J.A. -
Lecture 7: Atmospheric General Circulation
The climatological mean circulation Deviations from the climatological mean Synthesis: momentum and the jets Lecture 7: Atmospheric General Circulation Jonathon S. Wright [email protected] 11 April 2017 The climatological mean circulation Deviations from the climatological mean Synthesis: momentum and the jets The climatological mean circulation Zonal mean energy transport Zonal mean energy budget of the atmosphere The climatological mean and seasonal cycle Deviations from the climatological mean Transient and quasi-stationary eddies Eddy fluxes of heat, momentum, and moisture Eddies in the atmospheric circulation Synthesis: momentum and the jets Vorticity and Rossby waves Momentum fluxes Isentropic overturning circulation The climatological mean circulation Zonal mean energy transport Deviations from the climatological mean Zonal mean energy budget of the atmosphere Synthesis: momentum and the jets The climatological mean and seasonal cycle 400 Outgoing longwave radiation 350 net energy loss in Incoming solar radiation ] 2 − 300 the extratropics m 250 W [ x 200 u l f y 150 net energy gain g r e n 100 E in the tropics 50 0 90°S 60°S 30°S 0° 30°N 60°N 90°N 6 4 2 0 2 Energy flux [PW] 4 JRA-55 6 90°S 60°S 30°S 0° 30°N 60°N 90°N data from JRA-55 The climatological mean circulation Zonal mean energy transport Deviations from the climatological mean Zonal mean energy budget of the atmosphere Synthesis: momentum and the jets The climatological mean and seasonal cycle 400 Outgoing longwave radiation 350 Incoming solar radiation ] 2 − 300 -
Upwelling and Isolation in Oxygen-Depleted
Biogeosciences Discuss., doi:10.5194/bg-2016-34, 2016 Manuscript under review for journal Biogeosciences Published: 11 March 2016 c Author(s) 2016. CC-BY 3.0 License. Upwelling and isolation in oxygen-depleted anticyclonic modewater eddies and implications for nitrate cycling Johannes Karstensen1, Florian Schütte1, Alice Pietri2, Gerd Krahmann1, Björn Fiedler1, Damian Grundle1, Helena Hauss1, Arne Körtzinger1,3, Carolin R. Löscher1, Pierre Testor2, Nuno Viera4, Martin 5 Visbeck1,3 1GEOMAR, Helmholtz Zentrum für Ozeanforschung Kiel, Düsternbrooker Weg 20, 24105 Kiel, Germany 2LOCEAN, UMPC; Paris, France 3Kiel University, Kiel Germany 4Instituto Nacional de Desenvolvimento das Pescas (INDP), Cova de Inglesa, Mindelo, São Vicente, Cabo Verde 10 Correspondence to: Johannes Karstensen ([email protected]) Abstract. The physical (temperature, salinity, velocity) and biogeochemical (oxygen, nitrate) structure of an oxygen depleted coherent, baroclinic, anticyclonic mode-water eddy (ACME) is investigated using high-resolution autonomous glider and ship data. A distinct core with a diameter of about 70 km is found in the eddy, extending from about 60 to 200 m depth and. The core is occupied by fresh and 15 cold water with low oxygen and high nitrate concentrations, and bordered by local maxima in buoyancy frequency. Velocity and property gradient sections show vertical layering at the flanks and underneath the eddy characteristic for vertical propagation (to several hundred-meters depth) of near inertial internal waves (NIW) and confirmed by direct current measurements. A narrow region exists at the outer edge of the eddy where NIW can propagate downward. NIW phase speed and mean flow are of 20 similar magnitude and critical layer formation is expected to occur. -
Impact of Haida Eddies on Chlorophyll Distribution in the Eastern Gulf of Alaska
ARTICLE IN PRESS Deep-Sea Research II 52 (2005) 975–989 www.elsevier.com/locate/dsr2 Impact of Haida Eddies on chlorophyll distribution in the Eastern Gulf of Alaska William R. Crawforda,Ã, Peter J. Brickleyb, Tawnya D. Petersonc, Andrew C. Thomasb aInstitute of Ocean Sciences, Fisheries and Oceans Canada, P.O. Box 6000, Sidney, BC, Canada bSchool of Marine Sciences, University of Maine, Orono, Maine, USA cDepartment of Earth and Ocean Sciences, University of British Columbia, Vancouver, Canada Accepted 10 February2005 Available online 20 April 2005 Abstract Mesoscale Haida eddies influence the distribution of surface phytoplankton in the eastern Gulf of Alaska through two processes: enhanced productivityin central eddywater, and seaward advection of highlyproductive coastal waters in the outer rings of eddies. These two processes were observed in a sequence of monthlyimages over five years,for which images of SeaWiFS-derived chlorophyll distributions were overlaid by contours of mesoscale sea-surface height anomalyderived from TOPEX and ERS-2 satellite observations. Satellite measurements were supplemented with ship- based chlorophyll observations through one of the eddies. Haida eddies are deep, anticyclonic, mesoscale vortices that normallyform in winter and earlyspring near the southwest coast of the Queen Charlotte Islands. High levels of chlorophyll observed in eddy centres indicated that they supported phytoplankton blooms in spring of their natal years, with timing of these blooms varying from year to year and exceeding in magnitude the chlorophyll concentrations of surrounding water. Elevated chlorophyll levels also were observed in eddy centres in late summer and early autumn of their natal year. Enhanced chlorophyll biomass is attributed to higher levels of macro-nutrients and higher levels of iron enclosed within eddies than in surface, deep-ocean water. -
The Atmospheric General Circulation and Its Variability Dennis L
The Atmospheric General Circulation and its Variability Dennis L. Hartmann Department of Atmospheric Sciences, University of Washington, Seattle, Washington USA Abstract Progress in understanding the general circulation of the atmosphere during the past 25 years is reviewed. The relationships of eddy generation, propagation and dissipation to eddy momentum fluxes and mean zonal winds are now sufficiently understood that intuitive reasoning about momentum based on firm theoretical foundations is possible. Variability in the zonal-flow can now be understood as a process of eddy, zonal-flow interaction. The interaction of tropical overturning circulations driven by latent heating with extratropical wave-driven jets is becoming a fruitful and interesting area of study. Gravity waves have emerged as an important factor in the momentum budget of the general circulation and are now included in weather and climate models in parameterized form. Stationary planetary waves can largely be explained with linear theory. 1. Introduction: I attempt here to give a summary of progress in understanding the general circulation of the atmosphere in the past 25 years, in celebration of the 125th anniversary of the Meteorological Society of Japan. This is a daunting task, especially within the confines of an article of reasonable length. I will therefore concentrate on a few areas that seem most interesting and important to me and with which I am relatively familiar. I will not attempt to give a full list of references, but will attempt to pick out a few important early references and some more recent references. Interested readers can use these data points to fill in the intervening developments. -
Progress in Oceanography Progress in Oceanography 75 (2007) 287–303
Available online at www.sciencedirect.com Progress in Oceanography Progress in Oceanography 75 (2007) 287–303 www.elsevier.com/locate/pocean Mesoscale eddies dominate surface phytoplankton in northern Gulf of Alaska William R. Crawford a,*, Peter J. Brickley b, Andrew C. Thomas b a Institute of Ocean Science, Fisheries and Oceans Canada, P.O. Box 6000, Sidney BC, Canada V8L 4B2 b School of Marine Sciences, University of Maine, Orono, ME 04469-5741, USA Available online 31 August 2007 Abstract The HNLC waters of the Gulf of Alaska normally receive too little iron for primary productivity to draw down silicate and nitrate in surface waters, even in spring and summer. Our observations of chlorophyll sensed by SeaWiFS north of 54°N in pelagic waters (>500 m depth) of the gulf found that, on average, more than half of all surface chlorophyll was inside the 4 cm contours of anticyclonic mesoscale eddies (the ratio approaches 80% in spring months), yet these contours enclosed only 10% of the total surface area of pelagic waters in the gulf. Therefore, eddies dominate the chlorophyll and phytoplank- ton distribution in surface pelagic waters. We outline several eddy processes that enhance primary productivity. Eddies near the continental margin entrain nutrient – (and Fe) – rich and chlorophyll-rich coastal waters into their outer rings, advecting these waters into the basin interior to directly increase phytoplankton populations there. In addition, eddies carry excess nutrients and iron in their core waters into pelagic regions as they propagate away from the continental margin. As these anticyclonic eddies decay, their depressed isopycnals relax upward, injecting nutrients up toward the surface layer. -
Modeling the Generation of Haida Eddies
Modeling the Generation of Haida Eddies E. Di Lorenzo1, M.G. G. Foreman2, W.R. Crawford2 1Scripps Institution of Oceanography, University of California, San Diego, USA 2Institute of Ocean Sciences, Fisheries and Oceans Canada, Sidney, British Columbia, Canada Accepted for publication in Deep Sea Research II Revision January, 2004 1Corresponding author mailing address: Physical Oceanography Research Division Scripps Institution of Oceanography University of California, San Diego 9500 Gilman Drive , La Jolla, CA 92093-0213 Tel: (858) 534-6397 FAX: (858) 534-8561 e-mail: [email protected] FedEx address: 8810 Shellback Way, 439 Nierenberg Hall KEYWORDS: Gulf of Alaska, anticyclonic eddies, Haida eddy, buoyancy flows 1 Modeling the Generation of Haida Eddies E. Di Lorenzo1, M.G. G. Foreman2, W.R. Crawford2 1Scripps Institution of Oceanography, University of California, San Diego, USA 2Institute of Ocean Sciences, Fisheries and Oceans Canada, Sidney, British Columbia, Canada Abstract A numerical model forced with average annual cycles of climatological winds, surface heat flux, and temperature and salinity along the open boundaries is used to demonstrate that Haida Eddies are typically generated each winter off Cape St. James, at the southern tip of the Queen Charlotte Islands of western Canada. Annual cycles of sea surface elevation measured at coastal tide gauges and TOPEX/POSEIDON crossover locations are reproduced with reasonable accuracy. Model sensitivity studies show that Haida Eddies are baroclinic in nature and are generated by the merging of several smaller eddies that have been formed to the west of Cape St. James. The generation mechanism does not require the existence of instability processes and is associated with the mean advection of warmer and fresher water masses around the cape from Hecate Strait and from the southeast.