How Heterozygote Advantage Can Promote the Evolution of Disassortative Mating and Shape the Underlying Genetic Architecture?
Total Page:16
File Type:pdf, Size:1020Kb
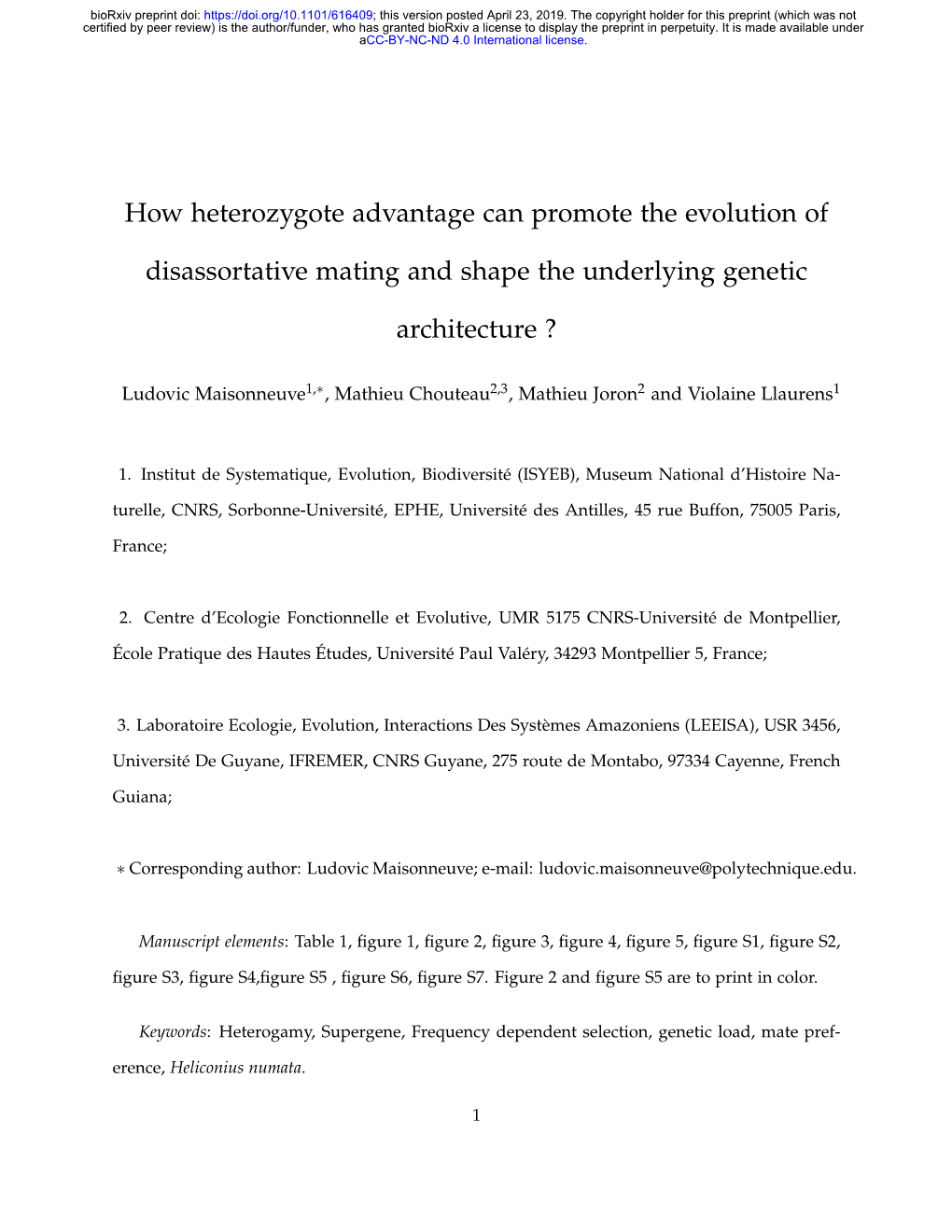
Load more
Recommended publications
-
Malaria and Sickle Cell Anemia
Malaria and Sickle Cell Anemia: Putting an Old Hypothesis to the Test Scientists have known for years that having sickled red blood cells can protect humans against malaria because sickled red blood cells are harder for the malaria-causing parasite to infect. Sickle cell trait, the phenotype associated with having one sickle cell allele and one normal allele (genotype H bAS) , is an example of heterozygote advantage because i n an environment where malaria is common, being homozygous in either direction—either having all sickled red blood cells (H bSS) or having all no sickled red blood cells (H bAA) —is a disadvantage. It is therefore u nsurprising that scientists have observed a high rate of sickle cell anemia among populations living in areas where malaria is very common. (After all, what happens when two carriers for sickle cell anemia have children together?) What might be surprising, however, is that although scientists have understood the importance of the link between malaria and sickle cell anemia for many years, it wasn’t until 2015 that a group of researchers set out to gather firsthand data. Before 2015, most of the data that scientists had used was taken from historical records of malaria prevalence. To get a more up-to-date picture, a group of scientists from the Research Institute for Development (IRD) in France undertook an epidemiological study t o observe the patterns of health and disease with relation to sickle cell anemia and malaria in the Republic of Gabon (see F igure 1 ). E pidemiology is a branch of medicine that studies the factors influencing health and disease in populations. -
Heterozygote Advantage Can Explain the Extraordinary Diversity of Immune Genes
bioRxiv preprint doi: https://doi.org/10.1101/347344; this version posted May 8, 2019. The copyright holder for this preprint (which was not certified by peer review) is the author/funder, who has granted bioRxiv a license to display the preprint in perpetuity. It is made available under aCC-BY 4.0 International license. Manuscript SUBMITTED TO eLife HeterOZYGOTE ADVANTAGE CAN EXPLAIN THE EXTRAORDINARY DIVERSITY OF IMMUNE GENES Mattias Siljestam1* AND Claus RuefflER1 *For CORRespondence: [email protected] (MS) 1Department OF Ecology AND Genetics, Animal Ecology, Uppsala University, Norbyvägen 18D, 752 36 Uppsala, Sweden AbstrACT The MAJORITY OF HIGHLY POLYMORPHIC GENES ARE RELATED TO IMMUNE FUNCTIONS AND WITH OVER 100 ALLELES WITHIN A POPULATION GENES OF THE MAJOR HISTOCOMPATIBILITY COMPLEX (MHC) ARE THE MOST POLYMORPHIC LOCI IN VERTEBRates. HoW SUCH EXTRAORDINARY POLYMORPHISM AROSE AND IS MAINTAINED IS CONTROversial. One POSSIBILITY IS HETEROZYGOTE ADVANTAGE WHICH CAN IN PRINCIPLE MAINTAIN ANY NUMBER OF ALLELES BUT BIOLOGICALLY EXPLICIT MODELS BASED ON THIS MECHANISM HAVE SO FAR FAILED TO RELIABLY PREDICT THE COEXISTENCE OF SIGNIfiCANTLY MORE THAN TEN alleles. WE PRESENT AN eco-eVOLUTIONARY MODEL SHOWING THAT EVOLUTION CAN IN A self-orGANISING PROCESS RESULT IN THE EMERGENCE OF MORE THAN 100 ALLELES MAINTAINED BY HETEROZYGOTE advantage. Thus, OUR MODEL SHOWS THAT HETEROZYGOTE ADVANTAGE IS A MUCH MORE POTENT FORCE IN EXPLAINING THE EXTRAORDINARY POLYMORPHISM FOUND AT MHC LOCI THAN CURRENTLY Recognised. Our MODEL APPLIES MORE GENERALLY AND CAN EXPLAIN POLYMORPHISM AT ANY GENE INVOLVED IN MULTIPLE FUNCTIONS IMPORTANT FOR survival. INTRODUCTION HeterOZYGOTE ADVANTAGE IS A WELL ESTABLISHED EXPLANATION FOR SINGLE LOCUS POLYMORPHISM WITH THE SICKLE CELL LOCUS AS A CLASSICAL TEXT BOOK EXAMPLE (Allison, 1954). -
Tay Sachs Disease Testing
Autosomal recessive mutations Genetic diseases & testing The story of three genetic diseases Phenylketonuria Reading: Chapter 12; and pp 201-202 Sickle-Cell Anemia Tay-Sachs Disease Phenylketonuria (PKU), Sickle-Cell Anemia and Tay-Sachs Disease are autosomal Frequencies of Sickle-Cell Anemia and Tay- recessive diseases. Sachs Disease alleles in different populations. carrier Disease is expressed in Sickle-Cell Anemia matings between carriers 10-40% of the population in regions of equatorial (heterozygotes). Africa are carriers <1% of South Africans are carriers Most affected individuals have unaffected parents. Tay-Sachs Increased frequency with 1/25 American Ashkenazi Jews are carriers inbreeding. 1/300 in the general population are carriers Why are the frequencies of some Hemoglobin disease alleles so high? Major protein in red blood cells. Hemoglobin is made of four Explanation #1 polypeptide chains--2 alpha and Heterozygote advantage 2 beta chains--and four heme- iron complexes. These complexes bind O2. Explanation #2 Founder effect Hemoglobin releases CO2 and binds O2 when CO2 concentrations are low. i.e., in the lungs. Hb binds CO2 and releases O2 when CO2 concentrations high. A single amino acid change in the beta peptide results in sickle cell anemia Why is the carrier frequency so high? Carriers have an advantage in malaria-infested areas Genotype disease malaria HbA/HbA normal susceptible HbA/HbS normal resistant Tay Sachs The Founder Effect Progressive disease with an onset in infancy of developmental retardation, followed by paralysis, dementia and blindness. Death occurs in the second or third year of life. Tay-Sachs disease is caused by mutation in the hexosaminidase A gene, which removes fatty substances called gangliosides. -
Pleiotropic Models of Quantitative Variation
Copyright 0 1990 by the Genetics Society of America Pleiotropic Modelsof Quantitative Variation N. H. Barton Department of Genetics and Biometry, University College London, London hW12HE, England Manuscript received May 23, 1989 Accepted for publication November 2 1, 1989 ABSTRACT It is widely held that each gene typically affects many characters, and thateach character is affected by many genes. Moreover, strong stabilizing selection cannot act on an indefinitely large number of independent traits. This makes it likely that heritable variation in any one trait is maintained as a side effect of polymorphisms which have nothing to do with selection on that trait. This paper examines the idea that variation is maintained as the pleiotropic side effect of either deleterious mutation, or balancing selection. If mutation is responsible, it must produce alleles which are only mildly deleterious (s = lo-'), but nevertheless have significant effects on the trait. Balancing selection can readily maintain high heritabilities; however, selection must be spread over many weakly selected polymor- phisms if large responses to artificial selection are to be possible. In both classes of pleiotropic model, extreme phenotypes are less fit, giving the appearance of stabilizing selection on the trait.However, it is shown that this effect isweak (of the same order as the selection on each gene): the strong stabilizing selection which is often observed is likely to be caused by correlations with a limited number of directly selected traits. Possible experiments for distinguishing the alternatives are discussed. HE main application of quantitative genetics to Following ROBERTSON(1967), we can contrast two T artificial and natural populations has been to kinds of explanation for these apparently contradic- use the pattern of genetic variances and covariances tory observations. -
In Drosophila Pseudoobscura*
A POPULATION CAGE TEST FOR HETEROSIS IN DROSOPHILA PSEUDOOBSCURA* JOHN A. SVED AND FRANCISCO J. AYALA The Rockefeller University, New York, N.Y. 10021 Received August 21, 1969 HE existence of high levels of heterozygosity in some natural populations, perhaps as high as 10-200/0 of the genome per individual, seems by now a well-established phenomenon (see review by SPIESS1968). Given the rather small suspected size of these populations, some explanation of how this variability is maintained seems necessary. In addition, as emphasized by LEWONTINand HUBBY(1 966), such explanation should take into account the high degree of similarity with respect to particular polymorphisms amongst populations, sug- gesting the likelihood that the polymorphisms are maintained by natural selection (see also PRAKASH,LEWONTIN and HUBBY1969). Heterozygote advantage, or heterosis, is one of the principal selective mecha- nisms at present under consideration. In the present article we will describe an experiment which is designed to investigate the adequacy of this explanation. Tests for heterosis: KING ( 1967) and SVED,REED and BODMER(1967) have recently emphasized the rather obvious point that an efficient method for testing whether the homozygote is usually at a disadvantage to the heterozygote is to compare the fitness of ordinary outbred individuals with that of individuals hav- ing a greater than normal proportion of homozygous loci, produced through some form of inbreeding. The results of many such tests are of course available, and it was argued that these results were compatible with only a limited amount of heterozygote advantage. A point which must be noted here is that tests such as the above give infonna- tion only on the overall homozygous disadvantage. -
Heterozygote Advantage for Fecundity Neil J
Heterozygote Advantage for Fecundity Neil J. Gemmell1*, Jon Slate2 1 School of Biological Sciences, University of Canterbury, Christchurch, New Zealand, 2 Department of Animal and Plant Sciences, University of Sheffield, Sheffield, United Kingdom Heterozygote advantage, or overdominance, remains a popular and persuasive explanation for the maintenance of genetic variation in natural populations in the face of selection. However, despite being first proposed more than 80 years ago, there remain few examples that fit the criteria for heterozygote advantage, all of which are associated with disease resistance and are maintained only in the presence of disease or other gene-by-environment interaction. Here we report five new examples of heterozygote advantage, based around polymorphisms in the BMP15 and GDF9 genes that affect female fecundity in domesticated sheep and are not reliant on disease for their maintenance. Five separate mutations in these members of the transforming growth factor b (TGFb) superfamily give phenotypes with fitness differentials characteristic of heterozygous advantage. In each case, one copy of the mutant allele increases ovulation rate, and ultimately litter size per ewe lambing, relative to the wildtype. However, homozygous ewes inheriting mutant alleles from both parents have impaired oocyte development and maturation, which results in small undeveloped ovaries and infertility. Using data collected over many years on ovulation rates, litter size, and lambing rates, we have calculated the equilibrium solution for each of these polymorphisms using standard population genetic theory. The predicted equilibrium frequencies obtained for these mutant alleles range from 0.11 to 0.23, which are amongst the highest yet reported for a polymorphism maintained by heterozygote advantage. -
The Eff'ects of Inbreeding at Loci with Heterozygote Advantage
THE EFF'ECTS OF INBREEDING AT LOCI WITH HETEROZYGOTE ADVANTAGE W. G. HILL AND ALAN ROBERTSON' Institute of Animal Genetics, Edinburgh 9 Received May 20, 1968 INCE the early studies of FISHERand WRIGHTthe theory of selection within populations of finite size has received much attention. KIMURA(1964) has reviewed the part of the theory that is based on continuous models in which it is usually assumed that individuals mate at random within small closed sub-popula- tions or lines. In such a situation we are concerned with the distribution of the frequency of individual genes over many replicate lines, or, equivalently, the distribution of the frequency of identical genes within the same line. In this report we study selection favouring heterozygous individuals with random mating within lines and no selection or crossing occurring between lines. The model for inbreeding which we discuss must be distinguished from an alternative situation, perhaps more common in plants, in which inbreeding occurs within an infinitely large population as a result of non-random mating, for example by selfing or mixed selfing and outcrossing. In the latter type of model, selection also may occur between sublines and recurrent mutation is not required for equilibria of gene frequency to occur without fixation, whereas it is in our model. These equilibrium situations have been analysed recently in some detail by ALLARD and co-workers. Many of their results for single loci are reviewed by JAINand WORKMAN(1967) and analysis of a two locus model is given by JAIN and ALLARD(1966). The effect of selection for heterozygous individuals in small lines when there is no between-line selection has been studied by REEVE(1955) using transition probability matrices for mating types in lines of only a few individuals, and by ROBERTSON(1962). -
Heterozygote Advantage As a Natural Consequence of Adaptation in Diploids Diamantis Sellisa, Benjamin J
Heterozygote advantage as a natural consequence of adaptation in diploids Diamantis Sellisa, Benjamin J. Callahanb, Dmitri A. Petrova,1, and Philipp W. Messera,1 Departments of aBiology and bApplied Physics, Stanford University, Stanford, CA 94305 Edited* by Boris I. Shraiman, University of California, Santa Barbara, CA, and approved November 2, 2011 (received for review September 7, 2011) Molecular adaptation is typically assumed to proceed by sequen- addition, expression-altering mutations are known to come in a tial fixation of beneficial mutations. In diploids, this picture variety of sizes ranging from subtle changes to dramatic ones of presupposes that for most adaptive mutations, the homozygotes tens of fold or even hundreds of fold (4, 5, 8). have a higher fitness than the heterozygotes. Here, we show that The model in Fig. 1, albeit instructive, may lack generality be- contrary to this expectation, a substantial proportion of adaptive cause it incorporates only a single trait. However, adaptation mutations should display heterozygote advantage. This feature of might often involve mutations that have complex pleiotropic adaptation in diploids emerges naturally from the primary impor- effects in an effectively multidimensional phenotypic space. The tance of the fitness of heterozygotes for the invasion of new classic model that incorporates this key feature of adaptation is adaptive mutations. We formalize this result in the framework of Fisher’s geometric model (9–13). In this single-locus model, Fisher’s influential geometric model of adaptation. We find that in phenotypes are vectors in an abstract geometric space (Fig. 2A), diploids, adaptation should often proceed through a succession of with the orthogonal axes representing different, independent short-lived balanced states that maintain substantially higher lev- traits, such as color and height. -
10.1534/Genetics.105.040683
Genetics: Published Articles Ahead of Print, published on May 23, 2005 as 10.1534/genetics.105.040683 The course of malaria in mice: MHC effects, but no general MHC heterozygote advantage in single-strain infections Claus Wedekind*,†, Mirjam Walker‡, §, ††, and Tom J. Little ** * Zoological Institute, University of Bern, 3012 Bern, Switzerland. † Program for Evolutionary Dynamics and Department of Organismic and Evolutionary Biology, Harvard University, Cambridge, MA, 02138, USA. ‡ Natural History Museum Bern, 3005 Bern, Switzerland. § Institute of Zoo and Wildlife Biology, 10252 Berlin, Germany. †† Harvard School of Public Health, Harvard University, Boston, MA 02115, USA. ** School of Biological Sciences, University of Edinburgh, Edinburgh EH9 3JT, Scotland, UK. - 1 - Running head: MHC and malaria in mice Keywords: MHC, malaria, heterozygote advantage, Plasmodium chabaudi, Correspondence to Claus Wedekind: Program for Evolutionary Dynamics, Harvard University, One Brattle Square, 6th floor, Cambridge, MA 02138, USA. E-mail: [email protected] - 2 - Summary A general MHC-heterozygote advantage in parasite-infected organisms is often assumed, although there is little experimental evidence for this. We tested the response of MHC- congenic mice (F2 segregants) to malaria and found the course of infection to be significantly influenced by MHC haplotype, parasite strain, and host gender. However, the MHC heterozygotes did worse than expected from the average response of the homozygotes. When fighting infection, MHC heterozygotes are often expected to be superior to both their respective homozygotes (i.e. exhibit overdominance) because they can present a wider range of antigens to T lymphocytes (DOHERTY and ZINKERNAGEL 1975). Moreover, MHC heterozygotes normally show slower disease progression and/or more rapid clearance of an infection than the average of homozygotes, further providing a population-level advantage of heterozygosity (e.g. -
NIH Public Access Author Manuscript New Phytol
NIH Public Access Author Manuscript New Phytol. Author manuscript; available in PMC 2014 January 09. NIH-PA Author ManuscriptPublished NIH-PA Author Manuscript in final edited NIH-PA Author Manuscript form as: New Phytol. 2014 January ; 201(1): . doi:10.1111/nph.12441. On the importance of balancing selection in plants Lynda F. Delph1,* and John K. Kelly2,* 1Department of Biology, Indiana University, 1001 East Third Street, Bloomington, IN 47405, USA 2Department of Ecology and Evolutionary Biology, University of Kansas, 1200 Sunnyside Avenue, Lawrence, KS 66045, USA Summary Balancing selection refers to a variety of selective regimes that maintain advantageous genetic diversity within populations. We review the history of the ideas regarding the types of selection that maintain such polymorphism in flowering plants, notably heterozygote advantage, negative frequency-dependent selection, and spatial heterogeneity. One shared feature of these mechanisms is that whether an allele is beneficial or detrimental is conditional on its frequency in the population. We highlight examples of balancing selection on a variety of discrete traits. These include the well-referenced case of self-incompatibility and recent evidence from species with nuclear-cytoplasmic gynodioecy, both of which exhibit trans-specific polymorphism, a hallmark of balancing selection. We also discuss and give examples of how spatial heterogeneity in particular, which is often thought unlikely to allow protected polymorphism, can maintain genetic variation in plants (which are rooted in place) as a result of microhabitat selection. Lastly, we discuss limitations of the protected polymorphism concept for quantitative traits, where selection can inflate the genetic variance without maintaining specific alleles indefinitely. -
Biological and Biomedical Implications of the Co-Evolution of Pathogens and Their Hosts
progress Biological and biomedical implications of the co-evolution of pathogens and their hosts Mark E.J. Woolhouse1, Joanne P. Webster2, Esteban Domingo3, Brian Charlesworth4 & Bruce R. Levin5 Co-evolution between host and pathogen is, in principle, a powerful determinant of the biology and genetics of infection and disease. Yet co-evolution has proven difficult to demonstrate rigorously in practice, and co-evolutionary thinking is only just beginning to inform medical or veterinary research in any meaningful way, even though it can have a major influence on how genetic variation in biomedically important traits is interpreted. Improving our understanding of the biomedical significance of co-evo- lution will require changing the way in which we look for it, complementing the phenomenological approach traditionally favored by evolutionary biologists with the exploitation of the extensive data becoming available on the molecular biology and molecular genetics of host–pathogen interactions. http://www.nature.com/naturegenetics That pathogens and hosts have evolutionary effects on one In this review, we assess the evidence for co-evolution in another and that these effects might be reciprocal—that is, host–pathogen systems. We then explore the complexities of pathogens and hosts co-evolve—are attractive, plausible and studying co-evolution in the ‘real world’, where there are many powerful ideas whose implications for the medical and veteri- different constraints on the potential for pathogen and host to nary sciences are only just beginning to be recognized1. An co-evolve. We proceed to consider the largely untapped oppor- important instance concerns the genetics of susceptibility to and tunity for studying co-evolution at the mechanistic as well as pathogenicity of infectious diseases. -
Host–Parasite Coevolution—Rapid Reciprocal Adaptation and Its
Zoology 119 (2016) 241–243 Contents lists available at ScienceDirect Zoology j ournal homepage: www.elsevier.com/locate/zool Editorial Host–parasite coevolution—rapid reciprocal adaptation and its genetic basis 1. Introduction scription factors containing the GATA motif, and in particular ELT-2, serve as key regulators for these immune responses. Coevolution between hosts and their parasites (here defined in a Within-generation plastic immunological regulation could cul- broad sense, including viruses, bacteria, fungi) provides fascinating minate in individualised immune responses, such as the highly examples of evolutionary adaptation. Host–parasite coevolution is specific immune memory in the vertebrate adaptive immune sys- predicted to be dynamic and rapid, mainly because fitness costs tems. Milutinovic´ et al. discuss accumulating evidence that not caused by parasites are high, parasites are ubiquitous, and they only vertebrates, but also invertebrates possess some kind of often evolve rapidly due to short generation times, large population immune memory, often denoted as ‘immune priming’ in arthro- sizes and flexible genomes. Studies of host–parasite coevolution pods. Priming subsumes a large set of potentially very diverse can thus provide powerful and versatile models for analysing the phenomena. Both the phenotypic characteristics and the mech- causes and processes of evolutionary adaptation more generally. anisms of priming seem to be highly divergent for different With the recent availability of new sequencing technologies, it host–pathogen combinations and for different routes of infection, has become possible to study ‘evolution in action’, i.e. the genetic which could limit the success of approaches based on candi- changes underpinning evolutionary processes. This approach is date genes.