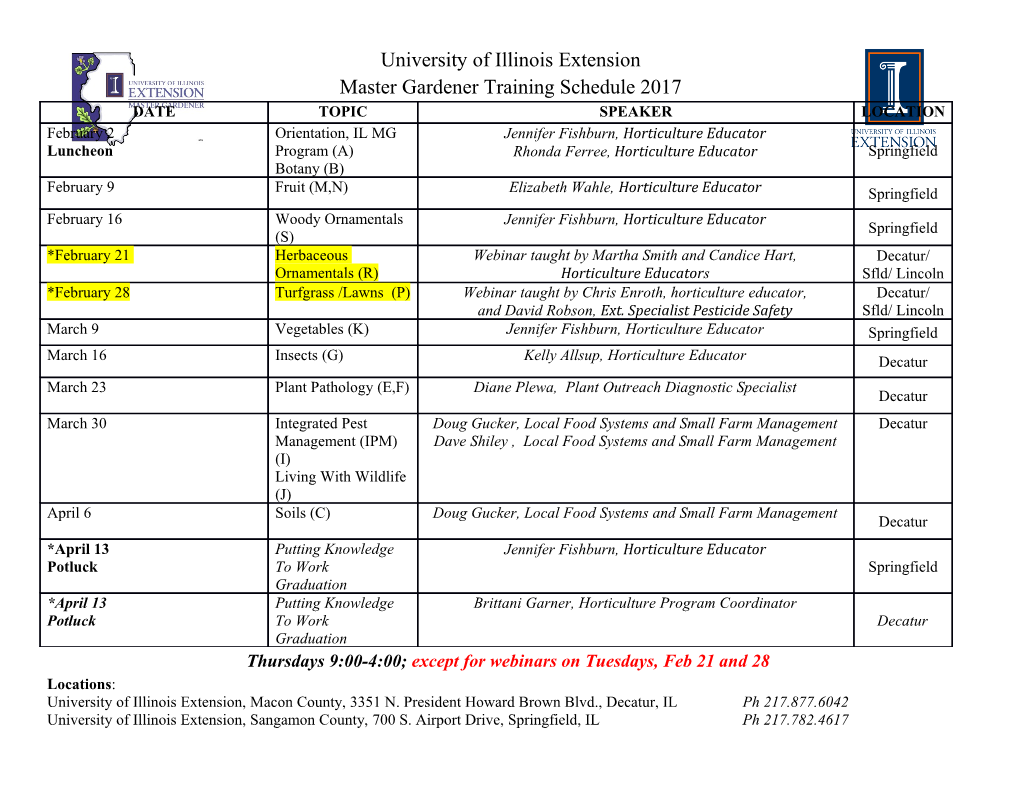
Rend. Sem. Mat. Univ. Pol. Torino Vol. 67, 2 (2009), 179 – 201 Second Conf. Pseudo-Differential Operators O. Liess and Y. Okada∗ THE KERNEL THEOREM INULTRADISTRIBUTIONS: MICROLOCAL REGULARITY OF THE KERNEL Dedicated to Professor Luigi Rodino on the occasion of his 60th birthday Abstract. In this paper we study kernels associated with continuous operators between spaces of Gevrey ultradistributions. The existence of such kernels has been established, in analogy with the kernel theorem of L. Schwartz for classical distributions, by H. Komatsu, and our aim here is to study these kernels from a microlocal point of view. The main re- sults, which are the theorems 2, 3 below, show that there is a significant difference between the results which hold true in the case of Beurling ultradistributions and the results valid for Roumieu ultradistributions. 1. Introduction The Schwartz kernel theorem states that the linear continuous operators T mapping D (U) to D ′(V) are precisely the operators for which there is K ∈ D ′(V ×U) such that (1) Tu(ϕ) = K (ϕ ⊗ u), u ∈ D (U), ϕ ∈ D (V ). (Cf. L. Schwartz, [17].) K is called the “kernel” of T and in this situation we write Rm Rn Tu(x) = U K (x,y)u(y)dy. Here U and V are open sets in and respectively, R ∞ ′ D (U) is the space of C 0 (U) functions endowed with the Schwartz topology and D (W) the space of distributions on W, with W = V or W = V ×U. The Schwartz theorem has been extended to the case of ultradistributions by H. Komatsu and both L. Schwartz and H. Komatsu have also studied linear continuous operators defined on compactly supported distributions, respectively ultradistributions, to distributions or ultradistri- butions. We shall consider for the moment only the distribution case. The problem is then to consider a linear continuous operator T : E ′(U) → D ′(V ), where E ′(U) is the space of compactly supported distributions on U. T induces a linear contin- uous operator on D (U) and therefore it has a distributional kernel K ∈ D ′(V ×U). The relation (1) associates a separately continuous bilinear form (ϕ,u) 7→ K (ϕ ⊗ u) on D (V ) × D (U) with T whereas the initial operator defined on E ′(U) is associated with the bilinear form (ϕ,u) 7→ T(u)(ϕ) defined on D (V ) × E ′(U). If we want to understand the class of kernels K ∈ D ′(V ×U) which correspond to linear continuous operators E ′(U) → D ′(V), we may then just study the bilinear form (ϕ,u) 7→ K (ϕ⊗u) as a form on D (V ) × E ′(U). This has led to a sophisticated theory of tensor products of topological vector spaces in which the notion of “nuclear” spaces (introduced by ∗The second author was supported in part by JSPS Grant-in-Aid No. 19540165. 179 180 O. Liess and Y. Okada A. Grothendieck) plays a central role. It turns out that most common spaces of dis- tributions or ultradistributions are nuclear and the central result concerning the kernel theorem in distributions is that the operator T : D (U) → D ′(V ) associated with some K ∈ D ′(V ×U) can be extended to a linear continuous operator E ′(U) → D ′(V ) if and only if K can be identified in a natural way with an element in [D (V )⊗ˆ E ′(U)]′, where D (V )⊗ˆ E ′(U) is, say, the ε topological tensor product of D (V ) with E ′(U). Since the spaces under consideration are nuclear, we may as well work with the π tensor product. For definitions and details we refer to [2] and [19]. There is also an interpretation of this in terms of C ∞ functions with distributional values. The theory of tensor products of topological vector spaces is very powerful and it explains, among other things, why kernel theorems in Banach spaces of (possibly generalized) functions must typically be more complicated than those in distributions (see e.g., [1] for some examples of kernel theorems in Lebesgue spaces): infinite di- mensional Banach spaces are never nuclear. On the other hand, when one wants to consider kernel theorems in hyperfunctions, this kind of approach is not usable in prac- tice since hyperfunctions have no reasonable topology. One may then try another ap- proach, which has been worked out in microlocal analysis. The central notion is this time the “wave front set” of a distribution, ultradistribution, or hyperfunction (intro- duced in 1969 by M. Sato for hyperfunction, [15] and in 1970 by L. Hörmander for distributions, [3]). The main condition is then (2) {(x,y,0,η);x ∈ V,y ∈ U,η 6= 0} ∩ WF(K ) = 0/. When K is a distribution, WF(K ) stands for the C ∞ wave front set and if (2) holds ′ then microlocal analysis gives a natural meaning to U K (x,y)u(y)dy when u ∈ E (U). (See [3], [20].) The same is true also in hyperfunRctions if WF denotes the analytic wave front set: there is a natural meaning for U K (x,y)u(y)dy when u is a real-analytic functional on U. Integration is then defined Rin terms of “integration along fibers” and U K (x,y)u(y)dy has a meaning in hyperfunctions: see e.g., [16], [5] for details. R There is now however a fundamental difference between the two main cases contemplated by microlocal analysis, the distributional and the hyperfunctional one. It is in fact not difficult to see that the condition (2) is not equivalent to the fact that K ∈ [D (V )⊗ˆ E ′(U)]′. This means that (2) is not a necessary condition when we want K to define a continuous operator from E ′(U) to D ′(V ). On the other hand, it is part of the results described in [10], [11], that for hyperfunctions a reasonable operator acting from some space of analytic functionals to the space of hyperfunctions can only be defined in presence of condition (2). It seemed then natural to the present authors to look into the case of Gevrey ultradistributions and to study if microlocal conditions of type (2) are necessary for reasonable operators in ultradistributions to exist. It came, at least at first, as a surprise, that the answer depends on which type of ultradistributions one is considering: for ultradistributions of Beurling type, one may work with weaker conditions than the ones corresponding to (2), whereas for ultradistributions of Roumieu type such conditions are also necessary: see section 2 for the terminology and the theorems 2, 3 for the precise statements. The kernel theorem in ultradistributions 181 2. Definitions and main results For the convenience of the reader, we shall now recall some of the definitions related to Gevrey-ultradistributions. (For most of the notions considered here, cf. e.g., Lions– Magenes, vol.3, section 1.3, or [14].) Consider s > 1, L > 0, U open in Rn and let K be a compact set in U. We shall denote by f 7→ | f |s,L,K the quasinorm |(∂/∂x)α f (x)| (3) | f | = sup sup , s,L,K |α| s α∈Nn x∈K L (α!) defined on C ∞(U). We further denote by • D s,L(K) the space of C ∞ functions f on Rn which vanish outside K such that for them | f |s,L,K < ∞, (s) s,L {s} s,L • D (K) = L>0 D (K),D (K) = L>0 D (K), T S {s} {s} (s) (s) • D (U) = K⊂U D (K), respectively D (U) = K⊂U D (K), S S (s) ∞ • E (U) = { f ∈ C (U); ∀K ⋐ U, ∀L > 0, | f |s,L,K < ∞}, respectively {s} ∞ E (U) = { f ∈ C (U); ∀K ⋐ U, ∃L > 0, | f |s,L,K < ∞}. The functions in E {s}(U), are called “ultradifferentiable” of Roumieu type, and those in E (s)(U), ultradifferentiable of Beurling type, with Gevrey index s. Since we shall often encounter statements for the two types of classes which are quite similar, we now introduce the convention that we shall write D ∗(U) when we give a statement which refers to both the case ∗ = (s) and the case ∗ = {s}. The same convention also applies for other spaces associated with the two cases. All the spaces mentioned above carry natural topologies: s,L • D (K) is a Banach space when endowed with |·|s,L,K as a norm, • D (s)(K) is the projective limit (for “L → 0+”) of the spaces D s,L(K), whereas D {s}(K) is the inductive limit (for “L → ∞”) of the same spaces. The spaces D (s)(K) are FS (i.e., Fréchet-Schwartz), whereas the spaces D {s}(K) are DFS (duals of Fréchet–Schwartz). (The topological properties of these spaces are studied in [6].) • D {s}(U) is the inductive limit (for K ⊂ U) of the spaces D {s}(K), whereas D (s)(U) is the inductive limit (again for K ⊂ U) of the spaces D (s)(K). • We shall define topologies on E (s)(U) and E {s}(U) as follows. At first we define ∞ for K ⋐ U and L > 0 the space YK,L of restrictions to K of functions in C (U), which satisfy | f |s,L,K < ∞, endowed with the topology given by the semi-norm |·|s,L,K . Then, E (s)(U) = lim limY , E {s}(U) = lim limY . ←− ←− K,L ←− −→ K,L K⋐U L>0 K⋐U L>0 182 O. Liess and Y. Okada We have continuous inclusions D ∗(U) ⊂ E ∗(U) with dense image and D ∗(K) is the subspace of E ∗(U) (K ⊂ U) consisting of the functions with compact support lying in K.
Details
-
File Typepdf
-
Upload Time-
-
Content LanguagesEnglish
-
Upload UserAnonymous/Not logged-in
-
File Pages23 Page
-
File Size-