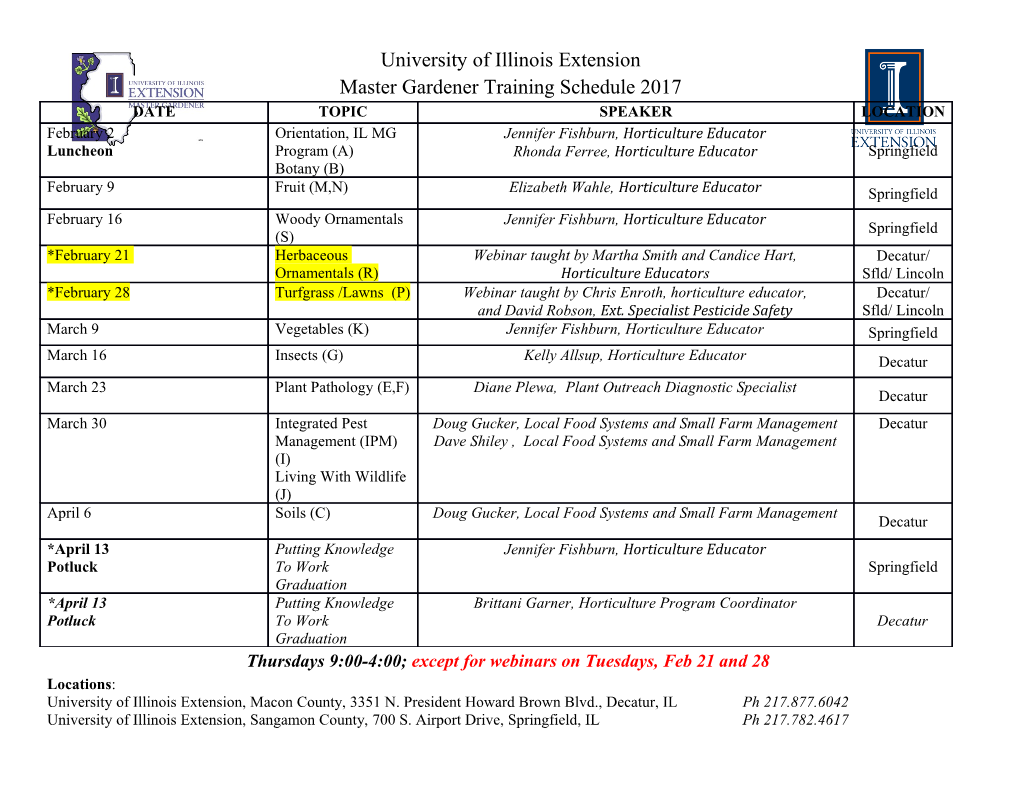
1 2 Structural Basis of Mitochondrial Dysfunction in Response to 3 Cytochrome c Phosphorylation at position 48 4 5 Blas Moreno-Beltrán1,*, Alejandra Guerra-Castellano1,*, Antonio Díaz-Quintana1, 6 Rebecca Del Conte2, Sofía M. García-Mauriño1, Sofía Díaz-Moreno3, Katiuska 7 González-Arzola1, Carlos Santos-Ocaña4, Adrián Velázquez-Campoy5, Miguel A. De la 8 Rosa1, Paola Turano2 & Irene Díaz-Moreno1,# 9 10 1Instituto de Investigaciones Químicas, cicCartuja, Universidad de Sevilla – CSIC, Avda. 11 Américo Vespucio 49, 41092 Seville (Spain). 12 2Magnetic Resonance Center (CERM) – Department of Chemistry, University of 13 Florence, Via Luigi Sacconi 6, 50019 Sesto Fiorentino, Florence (Italy). 14 3Diamond Light Source Ltd., Harwell Science and Innovation Campus, Didcot, 15 Oxfordshire OX11 0DE (United Kingdom). 16 4Centro Andaluz de Biología del Desarrollo, Universidad Pablo de Olavide – CSIC, and 17 CIBERER Instituto de Salud Carlos III, Carretera de Utrera km. 1, 41013 Seville (Spain). 18 5Institute of Biocomputation and Physics of Complex Systems (BIFI), Joint Unit BIFI- 19 IQFR (CSIC), Universidad de Zaragoza, Mariano Esquillor s/n, 50018 Zaragoza (Spain). 20 21 * These authors contribute equally to this work. 22 23 # Correspondence should be addressed to I. D.-M. ([email protected]) 24 Telephone number: +34 954489513; Fax number: +34 954460065 25 1 26 ABSTRACT 27 Regulation of mitochondrial activity allows cells to adapt to changing conditions and to 28 control oxidative stress, and its dysfunction can lead to hypoxia-dependent pathologies, 29 such as ischemia and cancer. Although cytochrome c phosphorylation—in particular, at 30 tyrosine 48—is a key modulator of mitochondrial signaling, its action and molecular basis 31 remain unknown. Here, we mimic phosphorylation of cytochrome c by replacing tyrosine 32 48 with p-carboxy-methyl-L-phenylalanine (pCMF). The NMR structure of the resulting 33 mutant reveals significant conformational shifts and enhanced dynamics around pCMF 34 that could explain changes observed in its functionality: the phosphomimetic mutation 35 impairs cytochrome c diffusion between respiratory complexes, enhances hemeprotein 36 peroxidase and ROS scavenging activities and hinders caspase-dependent apoptosis. 37 Our findings provide a framework to further investigate the modulation of mitochondrial 38 activity by phosphorylated cytochrome c and to develop novel therapeutic approaches 39 based on its pro-survival effects. 40 41 SIGNIFICANCE STATEMENT 42 Cell response to physiological changes and oxidative stress involves the modulation of 43 mitochondrial metabolism. Its dysfunction favors the development of hypoxia-dependent 44 pathologies, including ischemia and cancer. A key modulator of mitochondrial activity is 45 cytochrome c, whose cell function is modulated by tyrosine phosphorylation. However, 46 how such modification affects cytochrome c structure and function is barely known. Here, 47 we report that a phosphomimetic mutant of cytochrome c exhibits enhanced dynamics, 48 which could be responsible for the observed differences of cytochrome c functionality in 49 oxidative stress and cell death. Thus, phosphorylation of cytochrome c becomes a target 50 for further development of robust therapeutic approaches. 51 \body 2 52 INTRODUCTION 53 Oxidative phosphorylation (OxPhos) relies on the electron transport chain (ETC) to 54 generate the membrane potential that drives ATP synthesis (1). Components of the ETC 55 oxidize substrates to reduce molecular oxygen, thereby producing water. Nevertheless, 56 around 2% of the electrons flowing across the ETC yield reactive oxygen species (ROS) 57 (2). ROS are a source of oxidative stress and act as signaling molecules at low 58 concentrations (3-5). Hindering redox reactions within the distinct ETC membrane 59 complexes (complexes I to V) leads to enhanced ROS production (6). The activity of the 60 ETC is tightly regulated by posttranslational modifications of its components, isoform 61 swapping and modulation of the equilibria for the association of the membrane protein 62 complexes into supercomplexes (3, 7). Such associations allow substrate channeling 63 while modulating ROS production (3, 7). 64 Oxidative stress response involves redox signals modulating protein phosphorylation (8). 65 In the ETC, the major phosphorylation targets are NADH:UQ oxidoreductase, 66 cytochrome c (Cc) and cytochrome c oxidase (CcO) (9). Besides being essential for 67 oxidative respiration (Figure 1a), Cc acts as a redox regulatory protein within the 68 mitochondrial intermembrane space (10). In addition, Cc aids to control ROS levels by 69 oxidizing superoxide anions (11) and exhibiting peroxidase activity (12); the latter, 70 however, also yields lipid peroxidation (13, 14). Furthermore, Cc plays a crucial role in 71 programmed cell death, a process that is only in partially understood (15-21). In this 72 context, a fraction of Cc binds and oxidizes cardiolipin (CL) at the internal mitochondrial 73 membrane, thereby facilitating the release of unbound Cc to the cytoplasm (16, 17). In 74 mammalian cells, extramitochondrial Cc interacts with apoptosis activating factor-1 75 (Apaf-1) in the cytoplasm to spark the caspase proteolytic cascade (15). It has recently 76 been shown that Cc can interact with several other proteins outside the mitochondria in 77 humans and plants (18-21). The similarities between the Cc signaling networks in both 3 78 organisms suggest that key programmed cell death pathways are conserved along 79 evolution (22). 80 Cc phosphorylation is an alleged modulator of the mitochondrial cell death pathway (23, 81 24). Its deregulation is believed to be related to the onset of neurological disorders and 82 cancer (24). Phosphorylation of Cc is easily reversed by phosphatases, hampering the 83 isolation of the modified protein from tissues (25). Tyr-to-Glu substitutions of Cc, 84 designed to emulate Tyr48 phosphorylation, impair both the electron donation to CcO 85 and the activation of caspase-9 mediated by Apaf-1 in vitro (26, 27). Notably, nitration of 86 this residue also hinders the ability of Cc to activate Apaf-1 (28-30). Thus, these 87 posttranslational modifications alter both mitochondrial and cytoplasmic functions of Cc. 88 Tyr48 phosphorylation also affects the physical-chemical properties of Cc, such as its 89 alkaline transition and midpoint reduction potential (E0) (27, 31). Understanding the origin 90 of these effects and how they affect Cc activities requires to solve the 3D conformation 91 of the phosphorylated species. However, deciphering the effects of Tyr48 92 phosphorylation on the structure and dynamics of Cc is highly challenging because its 93 reactivity towards phosphatases. Indeed, no atomic resolution structure has been 94 reported for either phosphorylated Cc or any reliable mimic mutant. 95 Here, we have elucidated the structure of a phosphomimetic Cc variant in which Tyr48 96 is replaced by the synthetic, non-canonical amino-acid p-carboxy-methyl-L- 97 phenylalanine (pCMF). We show that such replacement induce local perturbations of the 98 Cc structure and enhanced internal dynamics of the mutation surroundings. We used 99 biochemical assays to show that the Y48pCMF mutation impairs Cc channeling between 100 cytochrome bc1 (Cbc1) and CcO, enhances the peroxidase activity and induces an anti- 101 apoptotic function of Cc. 102 103 104 RESULTS 4 105 Phosphorylation of Tyr48 induces local structural changes in cytochrome c 106 To understand how phosphorylation affects the structure of human Cc, we tackled the 107 challenge of fully characterizing the phosphomimetic mutant Y48pCMF Cc in its reduced 108 form, which is the redox state of Cc donating electrons to CcO in homeostasis and is 109 essential for its apoptotic activity, since Cc becomes highly reduced upon its release 110 from the mitochondria to the cytosol (32). Y48pCMF Cc maintains the overall secondary 111 structure and global fold of wild-type (WT) Cc, as inferred from circular dichroism (CD) 112 (Figure 1b) and 1H-15N heteronuclear single-quantum correlation (HSQC) nuclear 113 magnetic resonance (NMR) spectra (Figure 1c), respectively. Also, the native heme 114 axial coordination was preserved, as indicated by 1D 1H NMR data (Figure 1d). The 115 NMR spectra of reduced Y48pCMF Cc were extensively assigned: triple-resonance 116 experiments (Table S1) allowed us to detect and assign 91 backbone amide signals and 117 the sequential connectivities for most residues of the protein. Aliphatic side-chain signals 118 were assigned using 3D HBHA(CO)NH and 3D HCCH-TOCSY experiments, leading to 119 the assignment of most (96.4%) of the side-chain proton resonances. The 15N-H 120 resonance of pCMF48 was undetectable because the residue was not 15N-enriched. 121 Four proline residues (Pro30, Pro44, Pro71 and Pro76) interrupt the sequential HN-HN 122 connectivity. Additionally, in contrast to WT Cc, we could not detect the amide protons 123 of the Thr49, Ala51, Gly56 and Ile57 residues. However, the WT spectra also only had 124 four amide protons that could not be detected (Gly1, Glu21, Thr28 and Gly84) under 125 similar experimental conditions. Further, in contrast to WT Cc, the signals from Asn31, 126 Gly45 and Ser47 in pCMF48 Cc were significantly weaker than the rest. The largest 127 chemical-shift perturbations for backbone amides induced by the modified residue at 128 position 48 are confined to nearby residues (Figure S1). 129 Assigning 1H resonances to the heme substituents and 1H (and 13C) signals to the 130 aromatic side chains was required for analyzing additional 2D maps (i.e. COSY and 1H- 15 131 N NOESY) (Table S1). Assignment of the CCC and C aromatic signals from 5 132 the pCMF48 side-chain required the acquisition of an aromatic 1H-13C HSQC spectrum 133 recorded in a natural abundance of 13C (Figure 1e). The assignment of the pCMF48 134 side-chain signals involved the complete assignment of the 2D 1H-15N NOESY. 135 Numerous residues (Val20–Asn31, Thr40–Trp59 and Ile75–Glu90) displayed signals 136 attributable to a second, minor protein conformation, which had a 1:10 ratio in intensity. 137 Hereafter, only the major form was considered for structure calculations.
Details
-
File Typepdf
-
Upload Time-
-
Content LanguagesEnglish
-
Upload UserAnonymous/Not logged-in
-
File Pages76 Page
-
File Size-