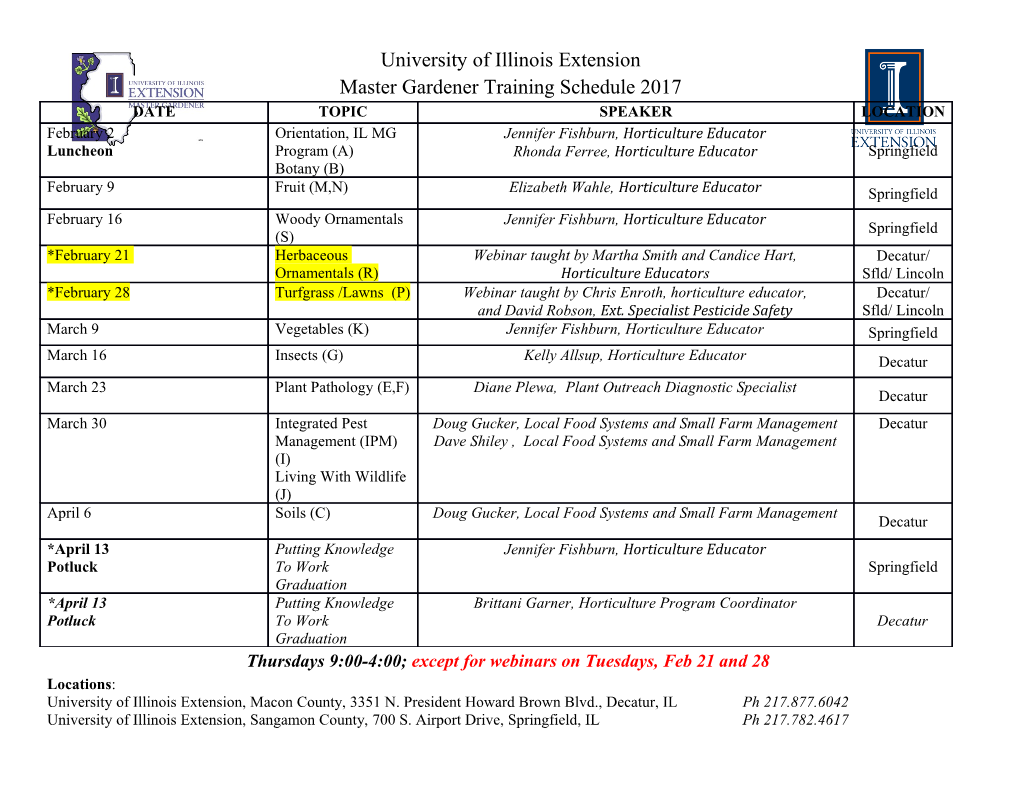
Experimental and Theoretical Studies on Mechanical Properties of Complex Oxides in Concrete By Juhyuk Moon A dissertation submitted in partial satisfaction of the requirements for the degree of Doctor of Philosophy in Engineering – Civil and Environmental Engineering in the Graduate Division of the University of California, Berkeley Committee in charge: Professor Paulo J.M. Monteiro, Chair Professor Shaofan Li Professor Hans-Rudolf Wenk Spring 2013 Abstract Experimental and Theoretical Studies on Mechanical Properties of Complex Oxides in Concrete by Juhyuk Moon Doctor of Philosophy in Engineering-Civil and Environmental Engineering University of California, Berkeley Professor Paulo J.M. Monteiro, Chair Despite the enormous amount of concrete consumed, fundamental understanding on the structural properties of hydrated oxides in concrete is still an open question. Due to the structural hierarchies and heterogeneous characteristics of concrete, accurate experimental and theoretical studies have not been well-developed for measuring mechanical properties of crystalline and amorphous materials in concrete. Lack of the information makes the development of constitutive relation of them to the macroscopic concrete properties difficult. The objective of this thesis is to compute mechanical properties of various phases in concrete. Unconventional methods of high pressure x-ray diffraction, absorption, and first-principles calculation are applied to calcium silicate hydrates (tobermorite 14 Å, 11 Å, 9 Å, and jennite), calcium aluminate hydrates (hemi- carboalumiante, monocarboaluminate, strätlingite, and hydrogarnet), tricalcium aluminate, and alkali-silica reaction gel. From the systematic comparison of both experiment and simulation, a comprehensive understanding of structural mechanism is achieved. For tobermorites, interlayer thickness which is related to the number of interlayer water molecules determines its compressibility. Hemicarboalumiante and strätlingite shows a pressure-induced dehydration while monocarboaluminate with the full occupancy of carbon oxide group behaves stable and incompressible under pressure. In the cases of tobermorite 14 Å and monocarboaluminate, linear density approximation in the first-principles calculation predicts the experimentally measured bulk modulus with high accuracy. Based on the excellent agreement of bulk moduli between experiment and simulation, it can be concluded that computed elastic properties of shear and Young’s modulus, 1 Poisson’s ratio, and elastic tensor coefficients are highly reliable. In addition, combining x-ray diffraction and absorption methods allows accurate measurement of density variation under pressure which is used to compute the bulk modulus of alkali-silica reaction gel. The fundamental structural properties obtained in both experiments and simulations given in this thesis will pave a path toward not just to increase our knowledge of the structural mechanism of concrete but also to optimize concrete design for better structural performance. 2 TABLE OF CONTENTS 1. Introduction..…………………………………………………………………….1 1.1 Research Motivation………………………………………………………..1 1.2 Outline of Thesis………………………………… ………………………5 2. Overview of Experimental and Theoretical Techniques………………………..6 2.1 Introduction to Synchrotron based x-ray experiments…………………6 2.2 First-Principles Calculation…………………………………………..11 2.3 Review on equations of state and micromechanics…………………..17 3. High Pressure X-ray Diffraction and First-Principles Calculation Studies on Calcium Silicate Hydrates………………………………… …………………..22 3.1 Calcium Silicate Hydrates in Concrete……………………………….22 3.2 High Pressure X-ray Diffraction Experiments on Calcium Silicate Hydrates.......................................................................................................28 3.2.1 Normal tobermorite 11Å……………………………………28 3.2.2 Anomalous tobermorite 11 Å…………………..……………36 3.2.3 Tobermorite 9 Å…………………………….……………….40 3.2.4 Jennite………………………………………………………43 3.3 First-Principles Calculations on Calcium Silicate Hydrates…….…….51 3.3.1 Tobermorite 14 Å…………………………………………...51 3.3.2 Tobermorite 9 Å…………………………………………….58 3.3.3 Jennite………………………………………………………62 3.4 Discussion on Structural Mechanism of Calcium Silicate Hydrates…68 3.5 Chapter Summary………………………………………………….….81 4. High Pressure X-ray Diffraction and First-Principles Calculation Studies on Calcium Aluminate Oxides……………………………………………………….83 4.1 Calcium Aluminate Hydrates in Concrete……………….……………83 4.2 High Pressure X-ray Diffraction Experiments on Calcium Aluminate Oxides…………..…………………………………………………….....86 4.2.1 Strätlingite……………………….…………………………..86 4.2.2 Hemicarboaluminate……………..…………………………..89 4.2.3 Monocarboaluminate………..……………………………….95 4.2.4 Tricalcium aluminate……………..………………………...100 4.3 First-principles Calculations on Calcium Aluminate Oxides…….….104 4.3.1 Monocarboaluminate……………………………………...104 4.3.2 Tricalcium aluminate…………………..…………………...115 4.4 Discussion on Elastic Properties of Calcium Aluminate Oxides and Calcium Aluminate Hydrated Oxides………………………………….119 4.5 Chapter Summary………………………….………………………...128 i 5. X-ray Absorption Experiments to Measure Pressure-induced Density Variation of Alkali-Silica Reaction Gel……………………………………………………130 5.1 Alkali-Silica Reaction Gel……………………….…………………..130 5.2 High Pressure X-ray Absorption Experiment on Alkali-Silica Reaction Gel………………….…………………………………………………….131 5.2.1 Chemical Compositions and Density of Alkali-Silica Reaction Gel……..……………………….………………....……………...131 5.2.2 Application of X-ray Absorption Method………………….133 5.3 Discussion on Measured Bulk Modulus of Alkali-Silica Reaction Gel………………………………………………………………….…….140 5.4 Chapter Summary……………………..…………………………...143 6. Conclusions…………………………………………………………………...144 References……………………………………………………………………….147 Appendix: Geometrically optimized atomic coordinates of crystals in concrete.162 ii LIST OF FIGURES Figure 1.1 Structural hierarchies in concrete. The images in nano and micro scales are from Richardson [2]. The concrete image in macro scale is from Mehta and Monteiro [1]. The atomic structure in a red box is tobermorite 14Å, reproduced from [3]………..……………………………………………………………….......2 Figure 2.1 Raw synchrotron x-ray powder diffraction patterns collected on (a) tobermorite 14 Å (Plombierite), (b) After heating the plombierite at 135 ºC for 1 day, (c) Pressurized at 6 GPa after the heating, and (d) synthesized C-S-H at ambient condition………………………………………………………………….8 Figure 2.2 Integrated synchrotron x-ray powder diffraction patterns of tobermorite 14 Å (Plombierite) at ambient condition, after heating at 135 ºC for 1 day, and pressurized at 6 GPa after the heating…………………………………………..…8 Figure 3.1 The analogy of x-ray diffraction patterns between synthesized C-S-H (red, bottom) and crystalline calcium silicate hydrates (normal tobermorite 11 Å and jennite)………………….……………………………………………………22 Figure 3.2 Schematic diagram showing the relative stability of part of the C-S-H compounds prepared under hydrothermal conditions [123]……………………...24 Figure 3.3 (a) The single dreierketten silicate structure in Hamid tobermorite 11 Å [129] (b) double (condensed) dreierketten silicate structure in Merlino tobermorite 11 Å [55]……………………………………………………………………….....25 Figure 3.4 (a) Silicate chains in tilleyite ribbon projected along [100] in jennite crystal structure. (b) Tilleyite ribbon projected along [010]. The silicate tetrahedra and calcium oxide octahedra are denoted in dark blue and light blue, respectively [118]........................................................................................................................25 Figure 3.5 Molecular model of C-S-H proposed by Pellenq et al. [142]. Silicon, calcium, oxygen, water molecules and hydroxyl groups are denoted with dark blue, light blue, red, white spheres, and black sticks, respectively.................................26 Figure 3.6 Crystal structure of tobermorite 11 Å projected along (a) [110] and (b) [010] proposed by Hamid [129]. Silicate chains, calcium octahedral, and oxygen atoms are shown as dark blue, light blue tetrahedra, and red spheres, respectively……………………………………………………………………….29 iii Figure 3.7 Crystal structure of tobermorite 11 Å projected along (a) [110] and (b) [010] proposed by Merlino et al. [55]. Same graphical notation in Fig. 3.1 is used.........................................................................................................................29 Figure 3.8 Variation of basal spacing of tobermorite 14 Å under heating……….32 Figure 3.9 Measured x-ray diffraction patterns of normal tobermorite 11 Å (λ = 0.6199 Å) with alcohol mixture (Methanol:ethanol=4:1). Bottom peaks indicate reference peak positions from [129]…………………………..………………….33 Figure 3.10 (a) Variation of axial lattice parameters of normal tobermorite 11 Å under pressure. (b) Refined unit cell volumes of normal tobermorite 11 Å under pressure. The 2nd and 3rd order BM EoS fittings give the bulk modulus of 71(4) GPa and 62(4) GPa……………………………………………………………….34 Figure 3.11 F-f plot of normal tobermorite 11 Å…………………………………35 Figure 3.12 Crystal structure of anomalous tobermorite 11 Å from Wessels mine, South Africa (a) and normal tobermorite 11 Å from Bashenov, Urals (b). The structures are projected along [010] and proposed by Merlino et al. [55, 116]. Same graphical notation in Fig. 3.1 is used……………………………………...36 Figure 3.13 Measured x-ray diffraction patterns of anomalous tobermorite 11 Å (λ = 0.6199 Å) with alcohol mixture (Methanol:ethanol=4:1). Bottom peaks indicate reference peak positions from [129]…………………………………………..…38
Details
-
File Typepdf
-
Upload Time-
-
Content LanguagesEnglish
-
Upload UserAnonymous/Not logged-in
-
File Pages191 Page
-
File Size-