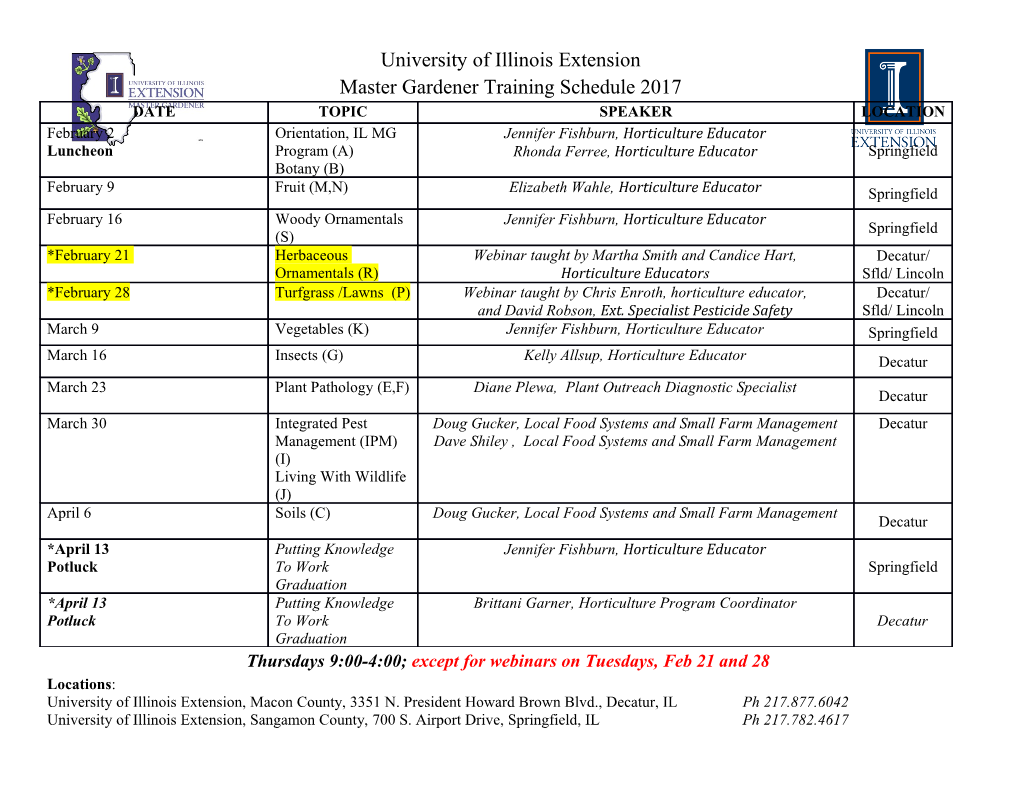
Development of Catalytic Reactor Designs for Enhanced CO Oxidation A Thesis submitted for the degree of Doctor of Philosophy in the University of London by Layla Kim Doory B.Sc. (Chemical Engineering) Ramsay Memorial Laboratory of Chemical Engineering, University College London, Torrington Place, London, WC1 7JE. ProQuest Number: 10609767 All rights reserved INFORMATION TO ALL USERS The quality of this reproduction is dependent upon the quality of the copy submitted. In the unlikely event that the author did not send a com plete manuscript and there are missing pages, these will be noted. Also, if material had to be removed, a note will indicate the deletion. uest ProQuest 10609767 Published by ProQuest LLC(2017). Copyright of the Dissertation is held by the Author. All rights reserved. This work is protected against unauthorized copying under Title 17, United States C ode Microform Edition © ProQuest LLC. ProQuest LLC. 789 East Eisenhower Parkway P.O. Box 1346 Ann Arbor, Ml 48106- 1346 ABSTRACT The catalytic removal of pollutants including nitrogen oxides (NOx), hydrocarbons (HC’s) and carbon monoxide (CO) in the exhaust of automobiles is generally performed by using monolithic supports coated with noble metal catalysts, notably platinum and rhodium (Pt/Rh) adsorbed onto a washcoat. This is typically achieved to within 90-98% conversion efficiency for average entry conditions (50<Re<400 at actual conditions), with pressure drops not exceeding 1.25-2 kN/m2. The monolith is a honeycomb structure, essentially composed of many parallel channels of square cross section. This therefore acts as a high surface area reactor. One of its drawbacks lies in the amount of precious metal requirements. With the increasing demand and price of these it is likely that in the future they may contribute to the cost of manufacture even more significantly. Detailed analysis shows that the overall rate of reaction of the monolith reactor is usually mass transfer rather than kinetically limited. Thus any boost in the mass transfer rate should increase conversion. Conversely for there to be any reduction in overall surface area or precious metal content there would have to be an increase in mass transfer rate. The effect of increasing mass transfer was studied by two methods namely by axially segmenting the ceramic monolith core sample (consisting of 62 cells/cm2 of 1.04 mm channels) and secondly by inserting static mixers into a catalyst coated pipe (ie."Active Transport Catalytic Reactor" (ATCR)). This was carried out for carbon monoxide oxidation over a commercially prepared catalyst supplied by Johnson Matthey. The intrinsic kinetics of this reaction were determined experimentally in a differential reactor. Conversions and pressure drops were measured for each system for varying Reynolds numbers from 73-440 (S.T.P.) in the channel and 160-2140 (S.T.P.) in the pipe, under stoichiometric reactant concentrations, and for steady state fully warmed up reactor conditions ranging from 250°C to 400°C. A one dimensional model is presented and its predictions compared to the experimental data for conversion and outlet gas temperature. Good agreement between experimental and theoretical data for the ATCR was found using the one-dimensional model for the conditions investigated. Also the model was found to be sufficiently accurate in predicting monolith conversions (ie. less than 10% difference between experiment and theory) and exit gas temperatures (ie. average of 4% difference) for high temperatures of 371°C and above. Pressure drops were also successfully predicted for both segmented monoliths as well as ATCR systems. Monolith segmentation was found to be successful in both enhancing CO oxidation as well as reducing the total catalyst requirements with the result that up to 30% saving of catalyst was possible. A simple optimization process using the theoretical data for the ATCR showed that up to 65% saving in reactor surface area (and hence catalyst requirements) is possible. Thus the novel idea of carrying out heterogeneous reactions within an ATCR shows promising results and indeed there is much scope for future research and possible applications. ACKNOWLEDGEMENTS I would like to extend my thanks to the following: Dr. S. P. Waldram for his supervision throughout this project and for his help in reading my thesis. Dr. U. Ullah for his kind help and for the use of the research facilities. Johnson Matthey for their help in supplying both the monolith cores as well as the catalyst coated tubes. SERC for the grant awarded to Dr. S. P. Waldram to support this reseach project. All the technicians for their assistance and help in building of the | equipment , in particular, Mr D. F. Montgomory, Mr K. W. Wheatley, Mr M.A Town, Mr J. S. Graham, Mr. M. Vale and Mr P. I. Punyer. Special thanks go to all my friends and colleagues within and out of the department who gave me constant support and encouragement. In particular I would like to thank Dr. L. Al-Dhahir for his kind and informative help in reading my thesis as well as the long and fruitful discussions throughout this project. Last but by no means least I would like to thank my parents for financing my education and for their continual love and support which they have provided throughout my period of study. To my loving father CONTENTS Page ABSRACT i ACKNOWLEDGEMENTS iii DEDICATION iv CONTENTS v NOMENCLATURE x CHAPTER 1. INTRODUCTION 1 1.1 Introduction 1 1.2 Objectives of the Study 3 CHAPTER 2. LITERATURE SURVEY 5 2.1 Introduction 5 2.2 Support Design 7 2.2.1 Types of support 7 2.2.2 Comparison of pellet and honeycomb converters 8 2.3 The Monolith 9 2.3.1 Description 9 2.3.2 Monolith variations 9 2.3.2.1 Material of construction 11 2.3.2.2 Channel size and shape 12 2.4 Types of Catalyst 15 2.4.1 Noble metal catalysts 15 2.4.2 Demand and supply of precious metals 16 2.4.3 Base metal catalysts 20 2.4.4 The washcoat 22 2.4.5 Promotors and stabilizers 23 2.5 Alternative Reactor Configurations 25 2.6 Kinetics of Heterogeneous CO Oxidation 28 2.6.1 Historical background 28 2.6.2 Multiplicities, hysteresis and self-sustained oscillations 31 2.6.3 The kinetic equation 33 v 2.7 The Overall Reaction Rate 34 2.8 Laminar Profile Within a Tubular Reactor 36 2.9 Reviews of Mathematical Models 40 2.9.1 Introduction 40 2.9.2 Monolith models 42 2.9.2.1 Low temperature operation 42 2.9.22 High temperature operation 53 2.9.23 Multiple steady states 57 2.9.2.4 Summary 59 2.9.3 Pressure drop in the monolith 61 2.9.4 The tubular flow reactor with static mixing elements 63 2.9.5 Pressure drop across the tubular and static mixer reactors 68 CHAPTER 3. PRELIMINARY EXPERIMENTAL SET-UP AND INVESTIGATION 73 3.1 Introduction 73 3.2 3.2.1 Apparatus 73 3.2.2 Gas analysis 78 3.3 Monolith Channel Size and Geometry 81 3.4 Procedures 82 3.5 Results and Discussion 84 3.6 Comments 91 CHAPTER 4. EXPERIMENTAL SET-UP 92 4.1 Introduction 92 4.2 Experimental Set-up 93 4.2.1 Gas supply and flow measurement 93 4.2.2 The pre-mixer/pre-heater 98 4.2.3 The reactor 99 * 4.2.4 Temperature measurement and control 99 4.2.5 Pressure drop measurement 101 4.2.6 Gas sampling and analysis 101 4.2.7 Data acquisition 102 4.3 Catalyst Pre-treatment 103 4.4 Experimental Technique 103 4.4.1 Procedures 103 v i 4.4.2 Sampling 104 4.4.3 Experimental error bounds on outlet conversion 104 4.4.4 Safety procedure 105 CHAPTER 5. THE KINETICS OF HETEROGENEOUS CO OXIDATION 106 5.1 Introduction 106 5.2 Differential Reactor Analysis 106 5.3 Kinetics of CO Oxidation on Pt/Rh Catalyst on g- 107 A120 3 5.3.1 The model 107 5.3.2 The observed kinetics 108 5.4 The Reactor 109 5.5 Procedures 112 5.6 Results and Discussion 114 5.6.1 Preliminary investigation 114 5.6.2 Reaction order with respect to each reactant 122 5.6.2.1 Check on reaction order wrt oxygen 122 5.6.2.2 Order wrt carbon monoxide 124 5.6.2.3 Solution method 125 5.7 Differential Reactor Conditions 126 CHAPTER 6. EXPERIMENTAL INVESTIGATION OF MONOLITH SYSTEMS 129 6.1 Introduction 129 6.2 The Reactor 129 6.3 Experimental Method 130 6.4 Results and Discussion 131 6.4.1 Effects of total inlet flowrate, Ft 131 6.4.2 Effect of of core length 138 6.4.3 Effect of number of segments 143 6.4.4 Effect of inlet temperature, Tcin 146 6.5 Enhancement Factors for Conversion 149 6.6 Conclusions 156 vii CHAPTER 7. EXPERIMENTAL STUDY OF THE TUBULAR AND ACTIVE TRANSPORT REACTORS 157 7.1 Introduction 157 7.2 The Reactor 157 7.3 Experimental Methods 162 7.4 Results and Discussion 162 7.4.1 The empty tubular reactor 162 7.4.1.1 Effect of flowrate 162 7.4.1.2 Effect of temperature 164 7.4.2 The ATCR 164 7.4.2.1 Effect of mixer configuration 164 7.4.2.2 Effect of number of mixers 171 7.5 Enhancement Factors for Conversion 171 7.6 Conclusions 177 CHAPTER 8 . COMPARISON OF EXPERIMENT WITH THEORY FOR THE MONOLITH AND ATCR SYSTEMS 179 8.1 Introduction 179 8.2 Model Assumptions 179 8.3 The One Dimensional Model 181 8.4 Solution Method 182 8.5 Choice of Sherwood and Nusselt Numbers for Use in the Model 183 8.6 Sherwood and Nusselt Numbers for the Monolith 186 8.7 Effect of Segmentation on Sherwood and Nusselt Numbers 186 8.8 Comparison of Experimental Conversion and Temperature Rise for the Monolith using the 1-D Model for Varying Inlet Temperature 189 8.9 Sherwood and Nusselt Numbers in the Active Transport and Tubular Reactors 195 8.10 Pressure Drop Predictions 203 8 .10.1 Pressure drop for the monolith 205 8.10.2 Pressure drop for the ATCR 205 v iii 8.11 Simplified Optimization of ATCR 209 8.12 Cost Estimates 217 8.13 Conclusions 217 CHAPTER 9.
Details
-
File Typepdf
-
Upload Time-
-
Content LanguagesEnglish
-
Upload UserAnonymous/Not logged-in
-
File Pages282 Page
-
File Size-