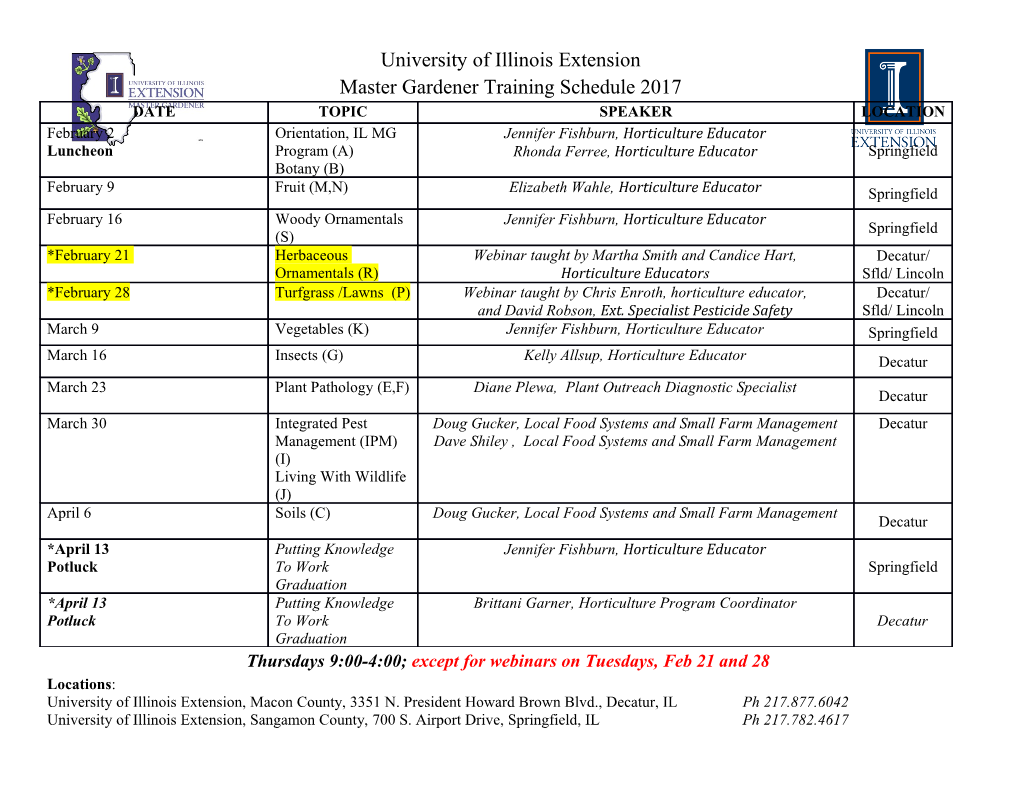
materials Review Inductive Thermal Effect of Ferrite Magnetic Nanoparticles Jeotikanta Mohapatra, Meiying Xing and J. Ping Liu * Department of Physics, University of Texas at Arlington, Arlington, TX 76019, USA; [email protected] (J.M.); [email protected] (M.X.) * Correspondence: [email protected]; Tel.: +1-817-272-2815 Received: 21 August 2019; Accepted: 24 September 2019; Published: 30 September 2019 Abstract: Localized heat induction using magnetic nanoparticles under an alternating magnetic field is an emerging technology applied in areas including, cancer treatment, thermally activated drug release and remote activation of cell functions. To enhance the induction heating efficiency of magnetic nanoparticles, the intrinsic and extrinsic magnetic parameters influencing the heating efficiency of magnetic nanoparticles should be effectively engineered. This review covers the recent progress in the optimization of magnetic properties of spinel ferrite nanoparticles for efficient heat induction. The key materials factors for efficient magnetic heating including size, shape, composition, inter/intra particle interactions are systematically discussed, from the growth mechanism, process control to chemical and magnetic properties manipulation. Keywords: iron oxide; magnetic nanoparticles; magnetic nanorods; nanocrystals nanoassemblies; superparamagnetism; colloidal stability; hyperthermia 1. Introduction Ferrite (MFe2O4,M = Mn, Fe, Co, Ni and Zn) magnetic nanoparticles (typically, of size 5–100 nm) have attracted profuse attention because they are at the interfaces of chemistry, physics and biology, due to their remarkable size and shape-dependent magnetic properties [1–3]. Scientists have developed methods to produce magnetic nanoparticles with fine control of the morphology [4–6]. Many new phenomena such as superparamagnetism, superferromagnetism, and superspin glass have been observed in these magnetic nanoparticles (MNPs) [7–9]. Moreover, in the nano-regime the magnetic properties such as coercivity (HC), saturation magnetization (MS) and susceptibility (χ) strongly vary with the size, shape, and composition of the magnetic nanoparticles [2,6,10]. These unique magnetic properties, small size and biocompatibility make them particularly promising in various biomedical applications, for instance contrast enhancement in magnetic resonance imaging (MRI), nano-sized carrier in drug delivery, mediators in converting electromagnetic energy to heat, and as magnetic-targeting and bio-sensing agents [11–15]. All these biomedical applications require the MNPs to have high magnetic moments with small sizes and a narrow particle-size distribution, so that the nanoparticles could have well-defined physical and chemical properties [11,16–18]. A general picture in Figure1 schematically illustrates the uses of MNPs in four important areas of cancer theranostics. In general, when the magnetic nanoparticle suspension is subjected to an alternating current magnetic field (ACMF), it can demonstrate prominent heating effects related to energy losses during the magnetization reversal of the nanostructures. This heating ability depends on the properties of the nanostructures, such as mean size, magnetization and magnetic anisotropy, and the ACMF amplitude (Hac) and frequency (f )[19,20]. Therefore, to improve the inductive heating characteristics of magnetic nanostructures, many approaches have been taken to improve the magnetic properties of the nanostructures [21–25]. Over the past few years, substantial progress has been made to precisely control the size, composition, morphology and multifunctionalities of magnetic nanostructures [11,26–29]. Materials 2019, 12, 3208; doi:10.3390/ma12193208 www.mdpi.com/journal/materials Materials 20172019,, 1012,, x 3208 FOR PEER REVIEW 2 2of of 31 30 nanostructures [11,26–29]. Such studies have improved our understanding of their unique nanoscale magneticSuch studies properties have improved and demonstrated our understanding the grea of theirt potential unique of nanoscale these MNPs magnetic in propertieshyperthermia and applications.demonstrated For the example, great potential MNPs of with these hard MNPs and in soft hyperthermia ferrite core-shell applications. architecture For example, exhibit strong MNPs exchange-coupling,with hard and soft ferritewhich core-shellenhances the architecture magnetic exhibitheating strong power exchange-coupling, [30,31]. The magnetic which nanostructure enhances comprisesthe magnetic of several heating domains power [30 such,31]. as The nanoflowers, magnetic nanostructure nanoclusters and comprises nanoassemblies of several have domains also been such shownas nanoflowers, to enhance nanoclusters the heating andpower nanoassemblies [24,25]. In this have review, also we been begin shown by tobriefly enhance introducing the heating the fundamentalpower [24,25 ].principles In this review, of MNPs. we beginWe then by focus briefly on introducing the chemical the synthesis, fundamental in particular principles the of organic MNPs. phaseWe then synthesis, focus on of the a series chemical of MNPs synthesis, with in controlled particular size, the organiccomposition, phase synthesis,and morphology. of a series Finally, of MNPs we establishwith controlled a relationship size, composition, across the magnetic and morphology. and inductive Finally, heating we establishproperties a of relationship MNPs. across the magnetic and inductive heating properties of MNPs. Figure 1.1. BasedBased onon the the heat heat activation activation ability, ability, these these nanostructures nanostructures have beenhave utilizedbeen utilized in cancer in therapy,cancer therapy,on-demand on-demand drug carrier drug and carrier gene and therapy gene applications therapy applications [32,33]. The [32,33]. magnetic The magnetic nanoparticles nanoparticles have also havebeen also as contrast been as agent contrast in magnetic agent in magnetic particle imaging particle (MPI)imaging and (MPI) magnetic and magnetic resonance resonance imaging (MRI).imaging (MRI). 2. Characteristics of Magnetic Nanoparticles and Inductive Heating Principles 2.2.1. Characteristics Magnetism at Nanoscaleof Magnetic Nanoparticles and Inductive Heating Principles 2.1. MagnetismAs first predicted at Nanoscale by Frenkel and Dorfman, when the size of the ferromagnet or ferrimagnet decreases below a critical size, the amount of energy required to produce domain walls becomes greater As first predicted by Frenkel and Dorfman, when the size of the ferromagnet or ferrimagnet than the reduction in the magnetostatic energy [34]. As a consequence, only single domain formation decreases below a critical size, the amount of energy required to produce domain walls becomes is favored. A single domain particle consists of large numbers of atomic spins and, thus, can be viewed greater than the reduction in the magnetostatic ener3gy [34].5 As a consequence, only single domain as ‘supermoment’ that has a magnetic moment ~10 to 10 µB [35]. The single domain size can be formation is favored. A single domain particle consists of large numbers of atomic spins and, thus, estimated from the equation [34–36]: can be viewed as ‘supermoment’ that has a magnetic moment1 ~103 to 105 µB [35]. The single domain 18(AK) 2 size can be estimated from the equation [34–36]:D = (1) SD 2 µ0MS Materials 2019, 12, 3208 3 of 30 whereMaterialsµ 2017is the, 10, permeabilityx FOR PEER REVIEW of free space (4π 10 7 H/m), A is the exchange stiffness in J/m3 of and 31 K 0 × − is the magnetocrystalline anisotropy in J/m3. For example, the typical value of single domain size is where μ0 is the permeability of free space (4π × 10−7 H/m), A is the exchange stiffness in J/m and K is around 128 nm for Fe O , while for MnFe O it is 50 nm (due to a smaller K and a higher M )[37–39]. the magnetocrystalline3 4 anisotropy in J/m23. 4For example, the typical value of single domainS size is Further, at the particle size d = DSD, the single domain prefers to be uniformly magnetized along one around 128 nm for Fe3O4, while for MnFe2O4 it is 50 nm (due to a smaller K and a higher MS) [37–39]. of its anisotropic easy axes, which leads to a strong enhancement in coercivity. Below DSD, due to the Further, at the particle size d = DSD, the single domain prefers to be uniformly magnetized along one decrease of the magnetic anisotropy energy (Ea = KV, V is volume of the particle) the coercivity value of its anisotropic easy axes, which leads to a strong enhancement in coercivity. Below DSD, due to the decreases with decrease in the size (see Figure2)[ 40]. On further reduction in size, the anisotropy decrease of the magnetic anisotropy energy (Ea = KV, V is volume of the particle) the coercivity value energydecreases value with decreases decrease further in the andsize becomes(see Figure comparable 2) [40]. On tofurther or even reduction lowerthan in size, the the thermal anisotropy energy (kBenergyT, kB is value Boltzmann decreases constant). further and As abecomes consequence, comparable the energy to or even barrier lower for magnetizationthan the thermal reversal energy is dominated(kBT, kB is byBoltzmann thermal constant). energy (Figure As a 3consequence,A, orange line). the Thus,energy the barrier supermoment for magnetization thermally reversal fluctuate is likedominated spins in aby paramagnetic thermal energy material, (Figure which 3A, orange leads toline). a net Thus, magnetization the supermoment of zero; thermally this phenomenon fluctuate is calledlike spins superparamagnetism.
Details
-
File Typepdf
-
Upload Time-
-
Content LanguagesEnglish
-
Upload UserAnonymous/Not logged-in
-
File Pages30 Page
-
File Size-