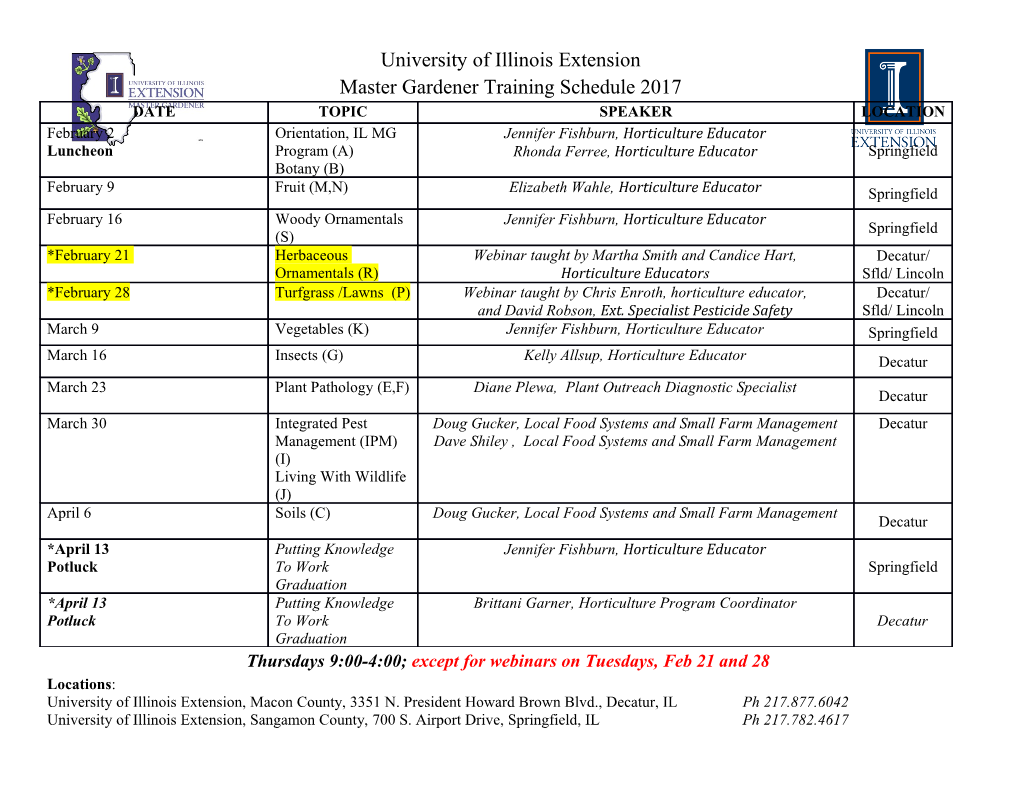
Surface Immobilization and Electrochemical Studies of Molecular Lanthanide and Actinide Complexes By Katie Jo Johnson B.S. Biochemistry, University of Oklahoma, 2015 Submitted to the graduate degree program in Chemistry and the Graduate Faculty of the University of Kansas in partial fulfillment of the requirements for the degree of Master of Science. Committee Members Dr. James D. Blakemore, Chair Dr. Kristin Bowman-James Dr. Mikhail V. Barybin Date Defended: 03/27/2019 The Thesis Committee for Katie J. Johnson certifies that this is the approved version of the following thesis: Surface Immobilization and Electrochemical Studies of Molecular Lanthanide and Actinide Complexes Committee Members Dr. James D. Blakemore, Chair Dr. Kristin Bowman-James Dr. Mikhail V. Barybin Date Approved: 04/04/2019 ii Abstract Due to their unique properties and emerging applications in materials, the chemistry of the lanthanides and actinides has become a thriving area of research. While much work has been done to probe the electronic, magnetic, luminescent, and catalytic properties of molecular lanthanide and actinide species, one area that has received less attention than it deserves is that of surface immobilization. The ability to successfully and selectively immobilize complexes on surfaces is crucial to the advancement of materials in fields such as sensor technologies, separations science, and catalysis. While most surface immobilized complexes contain d-block metals, this work focuses on the surface immobilization of complexes containing metals from the f-block. While Chapter 1 serves as a broad overview of the field of surface immobilization, Chapter 2 of this thesis discusses the synthesis and characterization of four new tripodal lanthanide complexes (Ln = Ce, Nd, Sm, Eu) capable of undergoing immobilization on graphitic surfaces. Characterization by NMR and XPS support that these lanthanide complexes retain their molecular structure in solution and after immobilization. Electrochemical studies of the redox active cerium complex allowed for the surface stability and interfacial electron transfer rates of the immobilized complex to be directly quantified. Chapter 3 of this thesis discusses the synthesis and characterization of two new molecular actinide species capable of noncovalent surface immobilization. Specifically, two new uranyl 2+ ([UO2] ) complexes have been synthesized and characterized by methods such as NMR and XRD. Following immobilization, electrochemical methods were used to probe the immobilized properties of these uranyl complexes using the presence of the U(VI/V) redox couple. These complexes demonstrated enhanced surface stabilities compared to the tripodal lanthanide complexes, as well as high rates of interfacial electron transfer. iii Table of Contents I. Approval Page ii II. Abstract iii III. List of Figures vii IV. List of Schemes xi V. List of Tables xiii VI. List of Abbreviations xiv Chapter 1: Introduction 1 1.1 An Overview of Surface Immobilization 2 1.2 Applications and Studies of Non-Covalent Surface Immobilization 6 1.3 Surface Immobilization of the f-Elements 14 1.4 Concluding Remarks 17 1.5 Thesis 18 1.6 References 20 Chapter 2: Noncovalent Immobilization of Molecular Lanthanide Complexes 29 2.1 Abstract 30 iv 2.2 Introduction 31 2.3 Results and Discussion 35 2.3.1 Ligand Synthesis 35 2.3.2 Synthesis and Characterization of M(LTP) Complexes 36 2.3.3 Surface Immobilization of M(LTP) Complexes 38 2.4 X-ray Photoelectron Spectroscopy (XPS) 38 2.4.1 Al Kα XPS Data 39 2.4.2 Mg Kα XPS Data 49 2.5 Cyclic Voltammetry 52 2.5.1 Stability Studies Utilizing Cyclic Voltammetry 57 2.5.2 Laviron Analysis and Rates of Electron Transfer 58 2.6 Conclusion 60 2.7 References 62 Chapter 3: Electrochemical Properties of Molecular Uranyl Complexes on Carbon Electrodes 68 3.1 Abstract 69 3.2 Introduction 70 3.3 Results and Discussion 75 2+ 3.4 Synthesis and Electrochemistry of [UO2] Salen Complexes 76 v 2+ 3.5 Synthesis and Electrochemistry of Pentadentate [UO2] Schiff base Complexes 86 3.6 Conclusion and Outlook 98 3.7 References 100 Chapter 4: Summary and Future Directions 108 4.1 Summary 109 4.2 Future Directions 114 ` 4.3 References 118 Appendix A: Supplemental Information for Chapter 2 122 Appendix B: Supplemental Information for Chapter 3 164 vi List of Figures Figure 1.1: Plot of the fractional coverage (Γ) versus time for the monopodal (2●3PF6, red) and tripodal (1●2PF6, blue) complexes from the Dichtel group. 10 Figure 1.2: Langmuir binding isotherms for a family of Co complexes at varying concentrations from the Dichtel group. 11 Figure 1.3: Fractional coverage (Γ/Γ0) versus time for a family of Co complexes from the Dichtel group. 12 Figure 1.4: Desorption kinetics of pyrene appended Ru complexes on HOPG electrodes from the Haga and Ozawa groups. 13 Figure 2.1: XP spectrum of a blank electrode 39 Figure 2.2: XP spectrum of LTP immobilized on a Ketjen black electrode 40 Figure 2.3: XP survey spectra for the M(LTP) complexes from Al Kα data 40 Figure 2.4: Stacked Al Kα source XP survey spectra for a blank electrode and electrodes functionalized with free LTP and M(LTP) complexes 41 Figure 2.5: Al Kα-source XP spectra for the N 1s region of the M(LTP) complexes 43 Figure 2.6: Al Kα source XP spectra for the respective M 3d regions for M(LTP) complexes 46 Figure 2.7: Al Kα source XP spectra for the respective M 4d regions for M(LTP) complexes 47 Figure 2.8: XP spectrum of a Kejen black electrode functionalized with free LTP utilizing the Mg Kα source 49 vii Figure 2.9: XP spectrum of the M 3d region of the immobilized Ce(LTP) complex utilizing the Mg Kα source 50 Figure 2.10: XP spectrum of the M 3d region of the immobilized Sm(LTP) complex utilizing the Mg Kα source 50 Figure 2.11: XP spectrum of the M 4d region of the immobilized Sm(LTP) complex utilizing the Mg Kα source 51 Figure 2.12: XP spectrum of the M 4d region of the immobilized Eu(LTP) complex utilizing the Mg Kα source 51 Figure 2.13: Cyclic voltammogram of the Ce(LTP) on a Ketjen black electrode 52 Figure 2.14: Cyclic voltammetry of Ce(LTP) and plot of ΔEp versus scan rate 53 Figure 2.15: Determination of anodic and cathodic peak current densities for all scan rates 55 Figure 2.16: Scan rate dependence voltammetry of the Ce(LTP) complex 55 Figure 2.17: Anodic and cathodic peak area ratios as a function of scan rate for Ce(LTP) 56 Figure 2.18: Fractional coverage plots for the Ce(LTP) and Ce(LTO) complexes 58 Figure 2.19: Laviron analysis plots for the Ce(LTO) and Ce(LTP) complexes 59 Figure 3.1: Contributions from various energy sources in the United States in 2017 70 Figure 3.2: Plot of the average atmospheric CO2 concentrations over time from 1958 to present 71 Figure 3.3: Cyclic voltammetry of the 1-U complex 78 viii Figure 3.4: Scan rate dependence studies of the 1-U complex 78 Figure 3.5: Cyclic voltammetry scans of the 1-U complex at various potential windows 79 Figure 3.6: Scan rate dependent studies done by Ikeda and co-workers on the uranyl salophen complex 81 Figure 3.7: Cyclic voltammetry of the immobilized 2-U complex 85 Figure 3.8: Cyclic voltammetry of the 3-U complex 87 Figure 3.9: Cyclic voltammetry studies at varying scan rates for the 3-U complex 88 Figure 3.10: Plot of the calculated ratios of the anodic and cathodic peak areas as a function of scan rate for 3-U 88 Figure 3.11: Enhanced 1H NMR spectrum of the 4-U complex’s aliphatic region 90 Figure 3.12: Cyclic voltammogram of the 4-U complex 91 Figure 3.13: Scan rate dependence of the 4-U complex 92 Figure 3.14: Plot of the calculated ratios of the anodic and cathodic peak areas as a function of scan rate for 4-U 93 Figure 3.15: Time-dependent cyclic voltammetry study of the 4-U complex 93 Figure 3.16: Comparison of the fractional coverage of the 4-U, Ce(LTP), and Ce(LTO) complexes determined from cyclic voltammetry 94 Figure 3.17: Plot of the anodic and cathodic peak currents as a function of scan rate for the 4-U complex 96 ix Figure 4.1: 1H NMR spectrum of the 5-U complex 117 x List of Schemes Scheme 1.1: Depiction of aromatic-aromatic interactions that give rise to π-π stacking geometries 5 Scheme 1.2: Depiction of the relationship between the dipoles and quadrupoles of a benzene molecule 5 Scheme 1.3: Depiction of a pyrene-appended nickel catalyst 6 Scheme 1.4: Depiction of the Co bis-terpyridyl complexes studied by the Dichtel group 9 Scheme 1.5: Depiction of the phenanthrene and naphthalene appended Co complexes reported by the Dichtel group 10 Scheme 1.6: Depiction of the pyrene-appended Ru complexes synthesized by the Haga and Ozawa groups 13 Scheme 1.7: Schematic representation of two systems used to immobilize lanthanide and actinide ions 15 Scheme 1.8: Schematic representation of a pyrene-appended Tb(III) complex 15 Scheme 2.1: Depiction of the Cp3Ln complex first synthesized by Wilkinson 31 Scheme 2.2: Common ligands used to encapsulate Ln(III) ions 32 Scheme 2.3: Representation of the M(LTO) and M(LTP) complexes 34 Scheme 2.4: Synthetic scheme for synthesis of compound 1 36 Scheme 2.5: Synthetic scheme for synthesis of the complete, tripodal LTP ligand 37 xi Scheme 2.6: Synthetic scheme for synthesis of M(LTP) complexes 37 Scheme 2.7: Color coded representation of the N 1s signals in the XPS spectra 43 Scheme 2.8: Diagram of the immobilized set-up used for electrochemical experiments 57 Scheme 3.1: Synthetic procedure to obtain the salen complex 1-U 76 Scheme 3.2: Depiction of a uranyl salophen complex studied by the Ikeada group 80 Scheme 3.3: Proposed electrochemical
Details
-
File Typepdf
-
Upload Time-
-
Content LanguagesEnglish
-
Upload UserAnonymous/Not logged-in
-
File Pages197 Page
-
File Size-