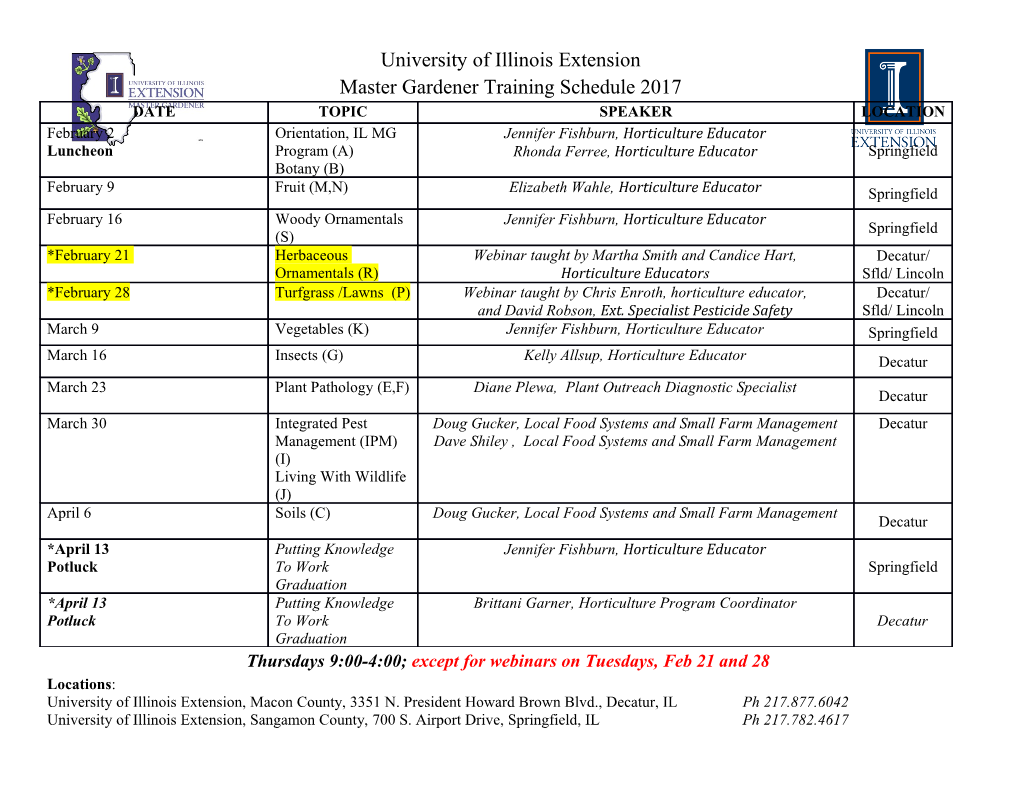
Chapter Two Ionization Energy Measurements Abstract The photorefractive effect depends strongly on the magnitude and rate of formation of the space-charge field. The space-charge field is created by the photogeneration, transport and trapping of charges. In polymers these processes are performed by specific molecules which are added to the material. Since these processes involve electron transfer from one molecule to another it is important to have information about the energy levels of the molecular orbitals of origin and destination. A technique is described which allows the determination of the HOMO energy levels of organic molecules. This technique, ultraviolet photoelectron spectroscopy (UPS), can be employed on molecules either in the gas or the solid phase. In this first attempt, the molecules were investigated in the gas phase, since only in this case can their properties be directly compared with each other. By doing so it was possible to obtain values for the HOMO levels of most of the molecules which are used in photorefractive polymers as described in the forthcoming chapters. Some preliminary understanding of the effect that certain molecules have upon the space-charge field formation and the photorefractive behaviour was obtained. 22 Chapter Two 2.1. Introduction The photorefractive mechanism can roughly be divided into two different regimes, the process of the space-charge field formation and the conversion of this space-charge field into a refractive index grating1. The latter process, although very important, will not be dealt with in this chapter but is thoroughly described in the next chapters. The process of the space-charge field formation is dependent on a number of parameters and can be described by: charge generation, charge transport and charge trapping, as was shown in chapter 1 (figure 1.2). There is one feature that all the processes involved in the space-charge field formation have in common, the transfer of an electron from one molecule to another. This can only be done if the electron overcomes the barrier between the two states2. The barrier that must be overcome depends on geometrical factors, the distance between the two molecules and the positioning of the molecules with respect to one another, but also on the energies of the state of origin and destination3. The total energies of the different states involved in the space-charge field formation are schematically presented in figure 2.1. Fig. 2.1: Schematic representation of the total energy of the states involved in the creation of a space-charge field. CG* and CG- represent the excited and the negatively charged charge generator molecule, CT*, CT and CT+ represent the excited, the neutral and the positively charged charge transport molecule and Tr+ represents the positively charged trapping site. It is possible to determine the energies of the different states if information about the ionization energy and electron affinity of the different molecules is available. The main molecular orbitals that are involved in the space-charge field formation are Ionization Energy Measurements 23 the highest occupied molecular orbitals (HOMO’s) of the charge generator, transport and trapping molecule and the lowest unoccupied molecular orbital (LUMO) of the charge generator molecule. One technique that gives information on the energy levels of occupied molecular orbitals is Photoelectron Spectroscopy (PES)4,5,6. In PES a molecule (M) is exited by a monochromatic beam of photons with energy hv, in which process M loses an electron. M + hv ® M+ + e (3.1) M+ is the resulting ion formed and e is the product photoelectron. In order for this process to occur, the incident photons should have an energy higher than the lowest ionization energy (EI) of the sample. It follows that the energy available after ionization, hv - Ip, must appear as translational energy of the electron. Thus, if mono-chromatic photons are used for ionization and the photon energy is known, a simple determination of the kinetic energy of the photoelectrons provides the ionization energy of the molecules. Depending on the energy of the photons employed, PES is sensitive to different energy ranges of molecular orbitals. For instance when x-ray sources are used to provide the photons, information about the core orbitals of the molecules and atoms under investigation is obtained. In this case the technique is referred to as XPS. When detailed information about the highest occupied molecular orbitals is needed, a source that provides photons with energies comparable to the first ionization energies of the sample is required. Usually a helium gas discharge lamp is used, which provides light in the vacuum-ultraviolet (VUV) region of the electromagnetic spectrum consequently the technique is referred to as Ultraviolet Photoelectron Spectroscopy (UPS). A schematic representation of this process is shown in figure 2.2. Here a molecule with five filled molecular orbitals is depicted, of which only three are accessible by the photons used in this experiment. Electrons can be ejected from these orbitals if photons are absorbed. This results in a molecular ion with three different final states, M+(1), M+(2) and M+(3), and three electrons with different kinetic energies. Usually the features are broadened by various vibrational relaxations. This is schematically depicted as the broadening of the lines in the photoelectron spectrum. 24 Chapter Two Fig. 2.2: Schematic representation of the processes involved in a UPS experiment. On the left, a molecule with five filled levels, three of which are accessible to the photons. In the middle: the molecular ions M+(1), M+(2) and M+(3) resulting from the ionization of the three highest occupied orbitals. On the right, the corresponding photoelectron spectrum reflecting molecular orbital levels is displayed. The peaks observed in this spectrum mimic the kinetic energy of the electrons. The energies of the originating molecular orbitals can now be reconstructed by subtracting the kinetic energy of the electrons from the known photon energy. For example, electrons ejected from the highest occupied molecular orbital (which will result in the creation of molecular ion M+(1)) will have the largest kinetic energy and will be observed as the first peak in the photoelectron spectrum. UPS can be performed both on molecules in the solid and in the gas phase6. The main difference between the UPS spectra of molecules measured in the solid and those measured in the gas phase is that in the solid phase interactions between neighbouring molecules play a significant role whereas they do not in the gas phase. These interactions between neighbouring molecules are mainly caused by their polarizabilities. When a molecule is photoionized it can be stabilized by interaction with induced dipoles on surrounding molecules. These interactions decrease the ionization energy and increase the electron affinity resulting in a decrease of the conductivity gap. From the above- described considerations it is not clear in which phase the molecules used in photorefractive polymers should be measured. If the investigated molecules would be used in the pure solid phase, it is obvious that the ionization energy should be measured in the solid phase. However, due to the fact that the molecules will be used in Ionization Energy Measurements 25 combination with an inactive polymer binder and, more importantly, with large concentrations of very polar NLO molecules, the values obtained from the pure solid phase will be inaccurate. This is caused by the difference in polarity of the actual surroundings and that of the investigated molecules, which will shift the energy levels of the investigated molecules, due to a change in dipolar interaction. For this reason the experiments were performed in the gas phase, as the effect of the surroundings is avoided and a more direct comparison of the energy levels can be made. The so obtained energy levels can only be used as an approximation to the actual situation if the highly polar environment has approximately the same effect on all the different molecules investigated. This assumption is a crucial one for the interpretation of the values obtained. 2.2. Experimental section 2.2.1. The ultraviolet photoelectron spectrometer The essential components of an ultraviolet photoelectron spectrometer are a lamp that produces suitable radiation, an ionization chamber, an electron energy analyser, an electron detector and a recorder5. These components are shown schematically in figure 2.3, and will be briefly discussed. Fig. 2.3: Essentials of an ultraviolet photoelectron spectrometer. All the electron optics must be contained within a vessel evacuated to 10-6 mbar or less. The lamp provides the photons which are used to ionize the sample. In order to reach the highest occupied orbitals, which generally have ionization energies between 26 Chapter Two five and fifteen electron volts, photons from a helium discharge lamp are used; more precisely the photons from the He I resonance line at 58.4 nm, equivalent to a photon energy of 21.22 eV. A hemispherical analyser, in combination with a lens system, was used as the electron energy analyser. This system was chosen because it provides a high resolution of the photoelectron spectrum5. Since the molecules are (large) organic molecules and solids at room temperature, some kind of heating device must be constructed to evaporate these molecules. In order to create a sufficiently high density for an acceptable signal-to-noise ratio, large amounts of molecules should be evaporated. Due to the organic nature of these molecules, however, they contaminate the apparatus, which prohibits accurate measurements due to charging effects. Therefore, a source was constructed which provides a collimated beam of gas molecules on which the helium lamp and the analyser are focused. In figure 2.4 a detailed drawing of the employed oven is shown. Fig. 2.4: A schematic representation of the source used to create a collimated beam of organic gas molecules.
Details
-
File Typepdf
-
Upload Time-
-
Content LanguagesEnglish
-
Upload UserAnonymous/Not logged-in
-
File Pages17 Page
-
File Size-